Exploring DNA to RNA Translation and Its Implications
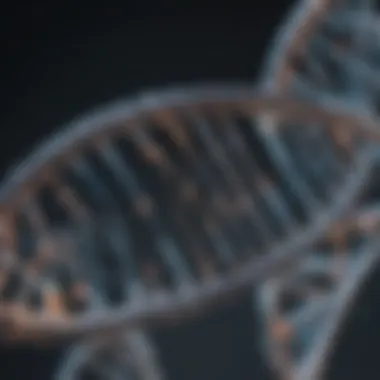
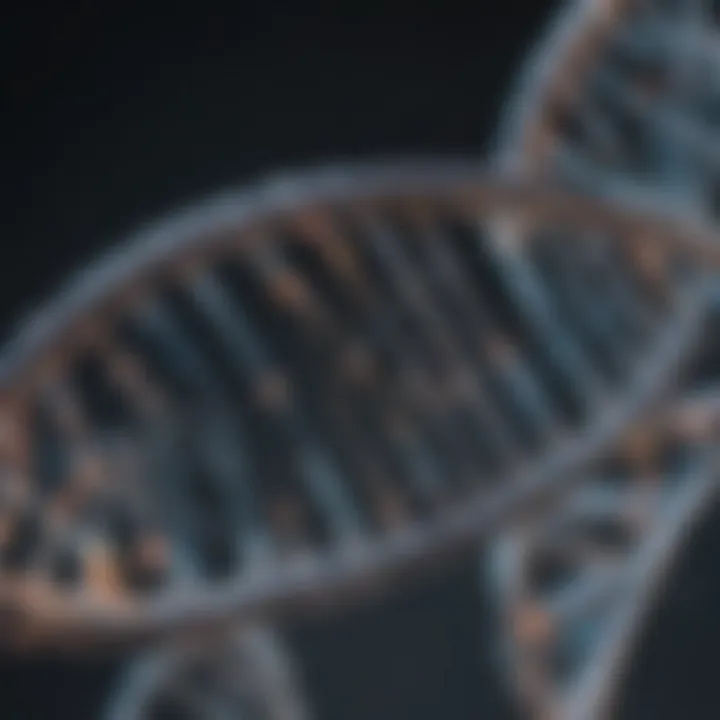
Intro
The process of translating DNA to RNA stands as a cornerstone of molecular biology, bridging the gap between genetic information and functional proteins. This intricate journey starts at the very essence of life: our genes, encoded in deoxyribonucleic acid (DNA). Understanding the transition from this stable, double-helix structure to its single-stranded counterpart, ribonucleic acid (RNA), is crucial. RNA serves not only as a messenger but also plays diverse roles in various biological processes.
Parsing through this complex translation requires not only a grasp on the underlying mechanisms but also an appreciation for the subtle nuances of gene expression. It’s essential to lay the groundwork by unraveling the components at play—enzymes, regulatory elements, and the molecular machinery involved. Delving into this topic can illuminate both the fundamental workings of life and its implications for advancements in fields like biotechnology and genetic engineering.
Methodology
Overview of Research Methods Used
The exploration of DNA to RNA translation encompasses diverse research methodologies aimed at unraveling the complexities of gene expression. Various techniques are instrumental in observing the processes at a cellular level.
- In vitro assays: These allow researchers to study transcription in a controlled environment, isolating the components necessary for RNA synthesis.
- Next-generation sequencing: This technology facilitates a comprehensive analysis of RNA profiles, thereby offering insights into gene expression patterns across different conditions and organisms.
- Bioinformatics tools: These are critical for analyzing vast amounts of data, often harnessing algorithms to decipher the intricate networks of gene regulation.
Data Collection Techniques
When it comes to studying the translation process, different data collection strategies provide pivotal insights.
- Experimental setups often involve manipulating variables such as temperature or enzyme concentrations, quantifying the resulting RNA output.
- Longitudinal studies in various organisms help elucidate evolutionary trajectories, revealing how transcriptional processes adapt over time.
- Quantitative RT-PCR is frequently used to measure the expression levels of specific genes, offering a snapshot of cellular responses under various conditions.
Understanding these methodologies is essential for grasping how scientists derive meaning from their data, providing a clearer picture of how DNA is ultimately translated into RNA.
Future Directions
Upcoming Trends in Research
As the field of molecular biology continues to evolve, several trends appear poised to shape future research on DNA to RNA translation:
- Single-cell RNA sequencing: This approach allows for the analysis of gene expression at unprecedented resolution, enlightening researchers on the heterogeneity within cell populations.
- CRISPR technology applications in transcription regulation have shown the potential to drive fine-tuned adjustments in gene expression profiles.
- Synthetic biology: Crafting custom RNA molecules for specific functions could lead to groundbreaking advancements in therapeutics and biotechnology.
Areas Requiring Further Investigation
Despite the wealth of knowledge acquired, there remains a multitude of avenues ripe for exploration:
- The interplay between transcription factors and regulatory elements needs deeper investigation to fully comprehend gene regulation intricacies.
- The role of non-coding RNAs in cellular processes is still not entirely understood and warrants further scrutiny.
- Finally, how epigenetic modifications influence the transcriptional landscape insists on detailed research, given their potential impacts on health and disease.
As our understanding of the translation from DNA to RNA deepens, so too does the horizon of scientific inquiry. By methodically exploring these themes, we not only enhance our comprehension of life at the molecular level but also open new doors for technological innovations, paving the way for future discoveries.
Understanding the Basics of Genetic Material
To truly grasp the ins and outs of how DNA translates to RNA, one must start by understanding the foundational elements that underpin genetic material. Without a firm grasp on the roles and characteristics of both DNA and RNA, the intricate processes that govern gene expression can seem daunting and, at times, opaque.
The Role of DNA
Deoxyribonucleic acid, commonly known as DNA, serves as the blueprint not just for an organism, but for life itself. The double helix structure, which looks like a twisted ladder, is not merely for show. Each rung of this ladder is made up of pairs of nitrogenous bases—adenine paired with thymine, and cytosine paired with guanine. This specific pairing is crucial because it ensures that genetic information is accurately transmitted during cellular replication.
The compact packaging of DNA in chromosomes is also vital. Each chromosome carries numerous genes, which are segments of DNA that encode for specific proteins. These proteins are the workhorses of the cell, facilitating countless processes essential for life. The role of DNA transcends mere storage of information; it is the very essence of heredity, passing traits from one generation to the next.
Moreover, DNA is subject to mutations, which are changes in the nucleotide sequence. While often the source of diversity in populations, some mutations can lead to genetic disorders or diseases.
The Function of RNA
Ribonucleic acid, or RNA, is the intermediary that translates the genetic blueprint found in DNA into the functional machinery of the cell. Unlike DNA, RNA is typically single-stranded, providing it with a degree of versatility. RNA comes in various forms, with messenger RNA (mRNA), transfer RNA (tRNA), and ribosomal RNA (rRNA) each playing distinct roles in the protein synthesis process.
- Messanger RNA (mRNA) serves as the template that carries the genetic code from DNA in the nucleus to the ribosomes, the cell's protein factories.
- Transfer RNA (tRNA) acts as the translator, bringing the correct amino acids to the ribosome during protein assembly.
- Ribosomal RNA (rRNA) contributes to the structural framework of ribosomes and even plays a catalytic role in protein synthesis.
The dynamic nature of RNA allows it to engage in various regulatory functions, including the recent discoveries around non-coding RNAs like microRNAs, which are involved in gene expression regulation. By providing a direct pathway for information flow, RNA takes the theoretical constructs of DNA and manifests them into tangible cellular functions.
"The elegance of genetic material is not just in its construction, but in its capacity to dictate the life processes across the spectrum of biological existence."
Understanding these two types of nucleic acids—DNA and RNA—sets the stage for a more nuanced exploration of the transcription process, through which genetic information transforms into functional products essential for life.
The Process of Transcription
Transcription is a fundamental phase in the flow of genetic information, connecting the rich code contained in DNA to the functional molecules of life—RNA. This process essentially serves as the blueprint for protein synthesis, which is crucial for various biological processes. Without transcription, the very fabric of cellular function would unravel; genes would remain dormant and proteins vital for life wouldn't be produced.
Understanding transcription is like holding the key to how our genetic information manifests. It's not merely a copy job; it's a carefully orchestrated procedure involving several intricate steps and crucial elements—each with its unique contribution to overall gene expression.
Initiation of Transcription
Promoter Recognition
At the outset of transcription lies promoter recognition, a pivotal step that sets the stage for RNA synthesis. Promoters are specialized DNA sequences located at the start of genes, acting as signposts for the transcription machinery. The unique feature of these promoter regions is that they contain specific motifs that are recognized by transcription factors. When these proteins bind to a promoter, they essentially signal to the entire transcription process that it’s game time.
What's beneficial about promoter recognition is its precision. This targeted approach ensures that only the right genes are activated at the right time, which is essential for cellular function. If we think about it, it’s like giving a green light to an important project only when all conditions are favorable. However, the downside is that mistakes in recognition can lead to improper gene expression, potentially resulting in diseases.
RNA Polymerase Binding
Following promoter recognition, RNA polymerase binding takes center stage. This enzyme is crucial because it’s the workhorse that synthesizes RNA based on the DNA template. RNA polymerase has a penchant for binding specifically to the promoter region, facilitated by transcription factors that form a bridge between the enzyme and the DNA.
The characteristic of RNA polymerase binding is its remarkable ability to unwind the DNA helix, allowing the genetic code to be transcribed into RNA. Such a feature is advantageous as it maintains the integrity of the template while launching the transcription process. One disadvantage, though, is that if RNA polymerase misbinds or disconnects prematurely, accuracy in RNA synthesis could be compromised, leading to truncated or erroneous RNA.
Elongation Phase
As the production of RNA progresses, we enter the elongation phase, where the real action unfolds. Here, RNA polymerase diligently adds nucleotides one by one to the growing RNA strand, matching each with its complementary DNA base. This phase is where the blueprint truly starts to take shape.
Nucleotide Addition
Nucleotide addition is the linchpin of the elongation phase. Each nucleotide, composed of a sugar, phosphate group, and nitrogenous base, is added in a sequence that mirrors the DNA template. The advantage of this process is that it enables rapid transcription of long RNA molecules. It's like assembling a complex LEGO model; as one piece clicks into place, the overall structure becomes clearer.
However, nucleotide addition can sometimes introduce errors. If a wrong nucleotide is incorporated, it might lead to mutations that could alter protein functionality. Such changes, if not corrected, might have rippling effects throughout cellular processes.
Template Strand Function
The template strand function is crucial in maintaining the fidelity of RNA synthesis. The DNA strand being read serves as the guide, ensuring that each added nucleotide matches correctly. This unique feature enhances the accuracy of the transcription process, as the complementary base pairing helps minimize errors.
That said, there is a complexity here; since there are two strands of DNA, mistakes in identifying which strand to read can lead to the wrong RNA transcript being generated. Such confusion could limit functional outcomes, complicating regulatory pathways in the cell.
Termination Mechanisms
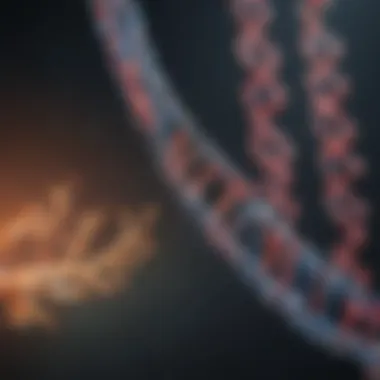
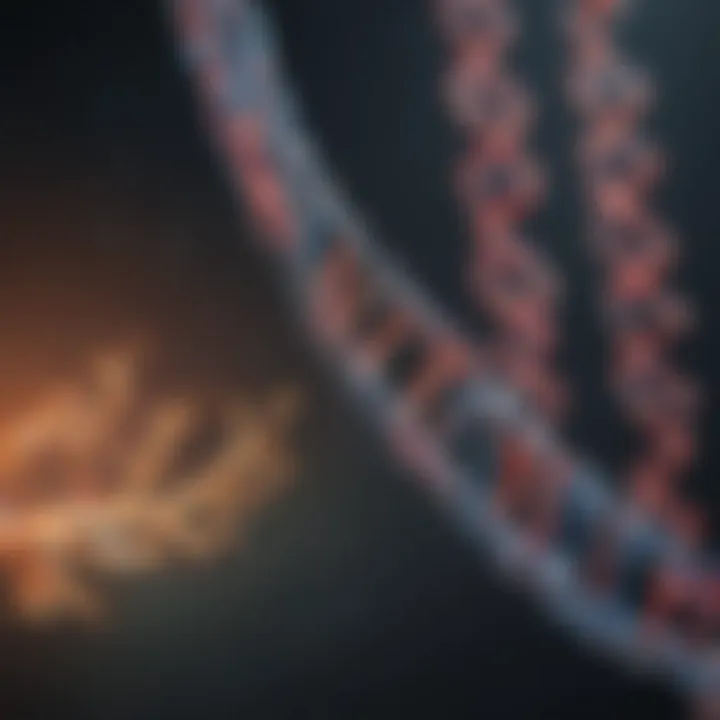
The process of transcription cannot simply go on indefinitely. As such, mechanisms of termination come into play to ensure a precise and efficient conclusion to RNA synthesis.
Signal Recognition
Signal recognition marks the end of RNA transcription when specific sequences indicate that the RNA polymerase should disengage. These signals are critical for ensuring that transcription halts at the appropriate location in the genome. One key characteristic of signal recognition is that it often involves intricate sequences that fold into structures recognized by the transcription machinery.
This is beneficial as it prevents unnecessary energy expenditure in producing excess RNA. However, if signals are too weak or mistimed, RNA polymerase may continue transcribing, potentially leading to extended RNA transcripts that disrupt cellular functions.
Termination Factors
Finally, termination factors are also pivotal in completing the transcription process. These proteins facilitate the disassembly of the transcription complex, allowing RNA polymerase to detach from the DNA. Their role is fundamental because they also play a part in processing the newly formed RNA, setting the stage for post-transcriptional modifications.
One unique feature of termination factors is their ability to respond to various cellular signals, making the transcription process adaptable. Still, there’s a disadvantage if these factors malfunction; improper termination could result in incomplete or erroneous RNA strands, which could contribute to malfunctioning cellular pathways.
Understanding the process of transcription in such detail not only enriches our grasp of gene expression but also opens paths for potential therapeutic interventions in cases where this elegant process goes awry. It’s clear that each step, from initiation to termination, is a crucial cog in the wheel of cellular machinery.
The Structure of RNA
Understanding the structure of RNA is crucial because it serves as the backbone for various processes that transfer genetic information from DNA to functional proteins. Unlike DNA, which often takes a double-helix form, RNA typically exists as a single strand that can fold into complex shapes, allowing it to perform different roles in the cell. At the core of its functionality is its chemical makeup, which includes a sugar-phosphate backbone and nitrogenous bases.
This structural versatility of RNA offers significant benefits for cellular processes. The ability to form different shapes means that RNA can interact with a variety of molecules, enhancing its role in gene regulation and protein synthesis. So, let’s delve into the various types of RNA and their unique contributions.
Types of RNA
mRNA
Messenger RNA (mRNA) serves as the intermediary that transmits genetic instructions from DNA to ribosomes for protein synthesis. Its main characteristic is the linear and unbranched structure, which allows it to carry the genetic code in a readable format. One important feature of mRNA is that it undergoes capping and polyadenylation before translation, which helps stabilize the molecule and facilitates its recognition by ribosomes.
In terms of advantages, mRNA plays a central role in protein synthesis, making it an essential aspect of the gene expression pathway. Its ability to encode specific proteins based on the sequence of nucleotides allows countless biological functions to be carried out in organisms, showing its popularity in the field of molecular biology. However, mRNA is also relatively short-lived, which means it needs to be constantly synthesized and degraded, a disadvantage in terms of stability.
tRNA
Transfer RNA (tRNA) acts as the adapter molecule in protein synthesis. It has a unique cloverleaf structure that allows it to carry specific amino acids to the growing polypeptide chain at the ribosome. A key characteristic of tRNA is the anticodon region, which is complementary to the mRNA codons, ensuring accurate translation of the genetic code into proteins.
One of the benefits of tRNA lies in its efficiency; it translates the three-nucleotide codons from mRNA into the corresponding amino acids, thereby facilitating the precision of protein synthesis. Nevertheless, its specificity means that each tRNA can only carry one type of amino acid at a time, which could be considered a limitation in some contexts.
rRNA
Ribosomal RNA (rRNA) is a primary component of ribosomes, the cellular machinery where proteins are synthesized. Its structure is notable for being the most abundant form of RNA, and it plays a critical role in maintaining ribosomal integrity and functionality. One specific characteristic of rRNA is that it can catalyze peptide bond formation, acting as a ribozyme, thereby demonstrating that RNA can have enzymatic activity.
The advantage of rRNA is its essential role in protein synthesis; without it, ribosomes could not function, and proteins could not be produced, which is critical for cellular life. On the downside, the sheer abundance of rRNA may lead to less focus during studies, as researchers often shift their attention to mRNA or tRNA in the context of gene expression.
Chemical Composition of RNA
The chemical composition of RNA provides vital insights into its various roles and mechanisms. It fundamentally consists of ribonucleotides, which are made up of three critical components: a nitrogenous base, a ribose sugar, and a phosphate group.
Nucleotide Structure
The structure of nucleotides is significant because it lays the groundwork for RNA functionality. Each nucleotide contains a ribose sugar rather than deoxyribose, which sets RNA apart from DNA. This difference gives RNA its unique properties. In terms of benefits, the presence of ribose contributes to RNA's ability to form versatile structures, making it more adaptable for various cellular roles. A downside, however, is that this ribose structure is less stable than deoxyribose, which can lead to RNA degradation over time.
Base Pairing Differences
Another critical aspect is how base pairing occurs in RNA. Unlike DNA, which uses thymine, RNA contains uracil as one of its nitrogenous bases, pairing with adenine during the formation of base pairs. This substitution is not merely a bit of trivia; it has implications for RNA's structure and function. The pairing differences allow RNA to form unique structures that are essential for its diverse roles, but this also limits the stability compared to DNA, potentially leading to more frequent mutations.
Understanding the structure of RNA not only enhances points of interest in molecular biology but also facilitates advancements in biotechnology and medicine.
Enzymes Involved in Transcription
In the process of transcription, enzymes play pivotal roles, ensuring that genetic information flows efficiently from DNA to RNA. This is not merely a mechanical task; it involves a finely-tuned dance of various proteins that work together to maintain the fidelity and regulation of gene expression. Understanding these enzymes provides insights into cellular functions and paves the path for advances in fields such as biotechnology and therapeutic developments.
RNA Polymerase
Types of RNA Polymerases
RNA polymerases are the key players in transcription, capable of synthesizing RNA from a DNA template. There are three main types of RNA polymerases in eukaryotic cells: RNA Polymerase I, II, and III. Each type serves distinct roles, thus contributing toward a well-orchestrated protein production process.
- RNA Polymerase I primarily handles the synthesis of ribosomal RNA (rRNA), which is crucial for ribosome function.
- RNA Polymerase II is responsible for producing messenger RNA (mRNA), the transcript that carries genetic information to the ribosome for protein synthesis. This enzyme's ability to recognize promoter regions in DNA is particularly significant, as it initiates the transcription of protein-coding genes.
- RNA Polymerase III deals mainly with transfer RNA (tRNA) and other small RNAs, playing a vital role in translating genetic code into functional proteins.
Each type's specialized function reveals a crucial aspect of gene regulation, allowing for diverse and dynamic control of biological processes. Thus, the unique and specific functionalities of different polymerases highlight their importance in cellular machinery.
Functional Role
The functional role of RNA polymerases extends beyond simply copying DNA into RNA. They also play roles in regulating gene expression by responding to various cellular signals.
The ability of RNA Polymerase II to interact with various transcription factors exemplifies this. These interactions allow for a nuanced response to environmental cues, thereby fine-tuning gene expression levels as per the cellular needs.
Another key function is the proofreading capability of RNA Polymerase. It can detect and correct errors during transcription, increasing the overall accuracy of RNA synthesis. This aspect is particularly beneficial, as it ensures that the genetic message remains intact and reduces potential mutation rates.
Other Essential Enzymes
While RNA polymerases are fundamental, they don’t act alone. Other essential enzymes also assist in various stages of transcription.
Helicases
Helicases are enzymes that unwound the DNA double helix, facilitating access for RNA polymerase to the template strand. Without helicases, the DNA strands would remain tightly coiled, prohibiting any interaction necessary for transcription.
These enzymes can speed up the process, as they unwind the DNA while RNA polymerase moves along the strand. This characteristic makes helicases invaluable in maintaining a consistent rate of transcription initiation and elongation. However, their activity needs to be carefully regulated; excessive unwinding could lead to genomic instability.
Topoisomerases
Topoisomerases provide another layer of support during transcription by addressing the supercoiling that occurs ahead of the RNA polymerase. When DNA unwinds, it naturally causes the strands to twist tighter elsewhere, potentially leading to tension.
- Type I topoisomerases cut one strand of DNA, allowing it to unwind and alleviate tension, while Type II topoisomerases cut both strands, ensuring smooth transcriptional flow.
- They are crucial for preventing DNA breakage, which could halt transcription and result in cellular malfunction.
In summary, the enzymatic machinery involved in transcription is an elegant system designed for precision and efficiency. Understanding these enzymes sheds light on the complexities of gene expression, and how any disruption in their functioning could lead to a cascade of cellular dysfunction.
"Enzymes in transcription are not just tools; they are orchestrators of genetic symphonies, guiding the creation of life itself."
This intricate dance of enzymes ensures that each step of the transcription process is regulated and efficient, allowing genetic information to be faithfully expressed in myriad forms, tailoring cellular functions across organisms.
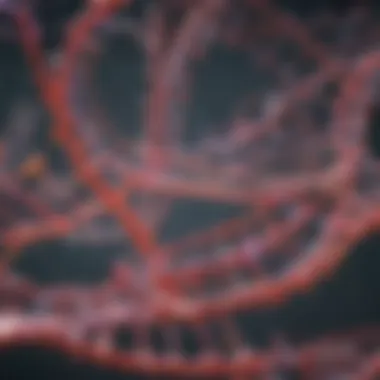
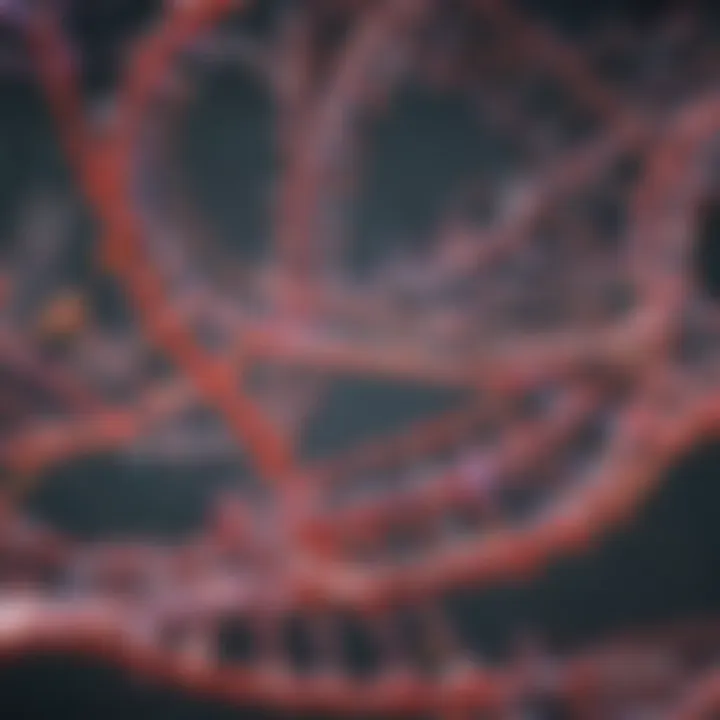
Regulatory Elements in Gene Expression
Understanding regulatory elements is key to unraveling the complexities of gene expression. These elements play a significant role in turning genes on or off, fine-tuning the process of transcription, and ultimately determining how proteins are synthesized. Without regulatory elements, genes might not function correctly, leading to a plethora of biological issues.
In the realm of gene expression, two major categories of regulatory elements come into play: promoters and enhancers, alongside silencers and insulators. Each of these components contributes uniquely to the precise control of gene activity, reflecting the intricate dance of molecular biology.
Promoters and Enhancers
Promoters are key players in the initiation of transcription. They serve as the binding sites for transcription factors and the RNA polymerase that kick-starts the transcription process. A typical promoter contains specific DNA sequences that are recognized by these important proteins. Thus, think of promoters as the launch pads where the transcription machinery takes off.
Enhancers, on the other hand, are regions of DNA that can significantly increase the transcription levels of associated genes. They can function over long distances, bending the DNA to bring the enhancer and promoter closer together. This may seem like a case of good teamwork, and indeed it is! By bridging gaps, enhancers enhance the interaction, leading to robust transcription activity.
Benefits of these regulatory elements include:
- Specificity: They help ensure that only relevant genes are activated in a particular cell type or under specific conditions.
- Flexibility: Genes can be activated or silenced in response to internal or external signals, like stress or hormonal changes.
- Coordination: Multiple enhancers can work together to ensure proper gene expression during development.
In essence, the relationship between promoters and enhancers is vital. They work in concert to modulate gene expression levels, significantly shaping cellular function and identity.
Silencers and Insulators
Silencers act as the opposite side of the regulatory coin. These elements can bind transcription factors that inhibit the transcription of target genes, ensuring that specific genes are silenced when they shouldn’t be active. This is crucial for maintaining the proper balance of gene activities within a cell, preventing unwanted expression that could lead to diseases, including cancer.
Insulators are unique as they function to maintain the boundaries between different regulatory domains. They can block the interaction between enhancers and promoters if they are not on the same regulatory domain.
Here's what makes silencers and insulators essential:
- Control of Overexpression: Silencers help to define the expression boundaries of genes, preventing potential overexpression that might disrupt cellular functions.
- Organizational Role: Insulators maintain gene regulatory architecture, ensuring that genes remain under the right control mechanisms.
- Genetic Stability: Both contribute to the overall genetic stability of an organism by controlling when and where genes are expressed.
"Gene expression is a beautifully orchestrated symphony where the regulatory elements are the skilled musicians that drive it forward."
By paying close attention to these nuances in regulatory elements, researchers can better grasp the complexities of gene expression and its implications in health and disease.
Post-Transcriptional Modifications
Post-transcriptional modifications play a pivotal role in the maturation of RNA molecules after transcription, ensuring that messenger RNA (mRNA) is properly processed before it can be translated into proteins. Understanding these modifications helps to unravel the complexities of gene expression and highlights the precision required for cellular function. This section will illuminate the significant elements of mRNA capping, polyadenylation, and RNA splicing, revealing their benefits and implications within the realm of molecular biology.
Capping of mRNA
The capping of mRNA is an essential modification that occurs at the 5’ end shortly after the initiation of transcription. A 7-methylguanylate cap (commonly referred to as a 'm7G cap') is added in a reverse orientation to the first nucleotide. This cap serves multiple purposes:
- Protection from degradation: The cap prevents exonucleases from degrading the mRNA strand, offering stability in the cytoplasm.
- Regulation of translation: The cap is recognized by the ribosome during the process of translation initiation, ensuring that the mRNA is efficiently translated into a protein.
- Facilitation of splicing: It also aids in the splicing processes later by participating in the nuclear export of mature mRNA.
Thus, the mRNA cap is not just a mere addition; it is a crucial element that enhances the reliability of gene expression.
Polyadenylation
Following transcription, a poly-A tail is added to the 3’ end of mRNA, a modification known as polyadenylation. This extension of adenine nucleotides contributes significantly to the stability and function of the mRNA:
- Enhances stability: The poly-A tail protects the mRNA from degradation in the cytoplasm, extending its lifespan.
- Facilitates translation: It promotes translation machinery recognition, further enhancing protein synthesis efficiency.
- Retroactive to transcription: Polyadenylation also signals the termination of transcription, marking the mRNA for further processing.
The poly-A tail is a classic example of how post-transcriptional modifications contribute to the overall utility of mRNA in cellular contexts.
RNA Splicing
RNA splicing is an intricate process whereby introns, or non-coding regions, are removed from the pre-mRNA transcript, and exons, or coding regions, are joined together. This process is vital for generating mature mRNA that accurately reflects the genetic code.
Introns and Exons
Introns and exons characterize eukaryotic genes, with introns acting as non-coding segments interspersed between the protein-coding exons. Exons contain the sequences that ultimately dictate the amino acid structure of proteins. The significance of this distinction resides in several aspects:
- Diversity of protein: By allowing alternative splicing of exons, a single gene can give rise to multiple proteins, thereby enhancing cellular diversity.
- Regulatory roles: Introns may harbor regulatory elements that influence gene expression and splicing efficiency, increasing the functional complexity of the gene's product.
- Precise splicing machinery: Exons' preparation for translation depends on them being spliced together accurately, emphasizing the importance of precision at this step.
Spliceosomes
Spliceosomes are large RNA-protein complexes essential for RNA splicing. They recognize splice sites on the pre-mRNA and catalyze the splicing reaction to remove introns. Key characteristics of spliceosomes include:
- Complexity and specificity: They consist of small nuclear RNAs (snRNAs) and multiple proteins, indicating a sophisticated structural assembly necessary for precise splice site recognition.
- Catalytic capability: The unique feature of spliceosomes lies in their ability to carry out the splicing reaction with great specificity, ensuring that only the correct introns are removed, and exons are joined effectively.
- Dynamic regulation: Spliceosomes also respond to various cellular signals, thus influencing alternative splicing events based on the cell's needs, showcasing the adaptability of gene expression.
In summary, post-transcriptional modifications, including capping, polyadenylation, and splicing, are critical processes that enhance mRNA's functionality and stability. These processes contribute to the dynamic regulation of gene expression and underscore how a single gene can lead to diverse protein products, fulfilling a multitude of roles in cellular biology. Through understanding these modifications, we gain insights into the broader mechanisms governing gene expression, which are essential in various contexts, from basic biology to applied biotechnology.
The Role of Non-Coding RNA
The burgeoning field of molecular biology has begun to shine a spotlight on non-coding RNAs, and for good reason. Unlike the well-known messenger RNAs that serve as templates for protein synthesis, non-coding RNAs (ncRNAs) play multifaceted roles in the intricate tapestry of cellular function. They may be small, but their impact is anything but trivial.
Non-coding RNAs can be thought of as the unsung heroes in the transcription landscape, contributing to gene regulation and maintaining genomic integrity without being directly translated into proteins. This section highlights specific elements, benefits, and considerations regarding non-coding RNAs, delving into two significant types: microRNAs and long non-coding RNAs.
"It’s not just about coding; sometimes what isn’t said speaks volumes."
MicroRNAs
MicroRNAs are a class of small, non-coding RNA molecules, often measuring around 21-24 nucleotides. They are pivotal in regulating gene expression post-transcriptionally. This regulation occurs when microRNAs bind to complementary sequences on target mRNAs, typically resulting in mRNA degradation or the inhibition of translation. In other words, microRNAs determine the fate of various genes, which can have extensive implications for cellular activities.
Some critical aspects regarding microRNAs include:
- Gene Regulation: They can modulate over half of all human genes, illustrating their extensive influence on cellular functions. This includes roles in development and differentiation, apoptosis, and responses to stress.
- Role in Disease: Aberrant microRNA expression has been implicated in various diseases, particularly cancer. For instance, certain microRNAs can function as tumor suppressors or oncogenes, complicating therapeutic strategies.
- Potential Biomarkers: Due to their stability in bodily fluids, microRNAs can be investigated as biomarkers for disease diagnosis and prognosis. Their presence or absence in different conditions may provide insights into health status.
Long Non-Coding RNAs
Now, moving on to long non-coding RNAs, which generally exceed 200 nucleotides in length. While they remain less understood than microRNAs, their emerging importance is clear. These molecules assist in a wide range of cellular processes including chromatin remodeling, transcriptional control, and even splicing patterns. Instead of being directly translated, they engage in more complex interactions within the cell.
Important features of long non-coding RNAs include:
- Chromatin Regulation: Many lncRNAs have been shown to interact with chromatin-modifying complexes, facilitating gene expression silencing or activation. This function is pivotal in maintaining cellular identity and response to environmental signals.
- Cellular Localization: They can influence gene expression through their presence in specific cellular compartments. For example, some lncRNAs are localized in the nucleus and exhibit roles in gene transcription regulation.
- Interaction with RNA-binding Proteins: Long non-coding RNAs can serve as scaffolds for various proteins, impacting their activity and cellular outcomes. This indicates a robust network of interactions that can dictate cellular fates.
In summary, non-coding RNAs, thriving in the shadows of genetic understanding, are indispensable to cellular function and regulation. They provide mechanisms that fine-tune gene expression and maintain the delicate balance necessary for life. As research continues, the breadth of knowledge surrounding these molecules will expand, revealing untapped therapeutic potential and a deeper understanding of genetic regulation.
Implications of RNA Translation in Cellular Function
Understanding how RNA is translated from DNA is just the tip of the iceberg when it comes to grasping its significance in cellular function. The implications of RNA translation stretch far beyond the basic rules of molecular biology. They encompass how genes are regulated, how cellular processes are executed, and how these factors contribute to the functioning of living organisms.
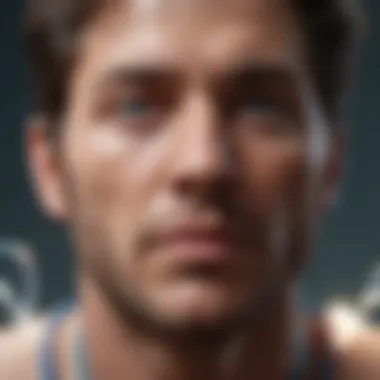
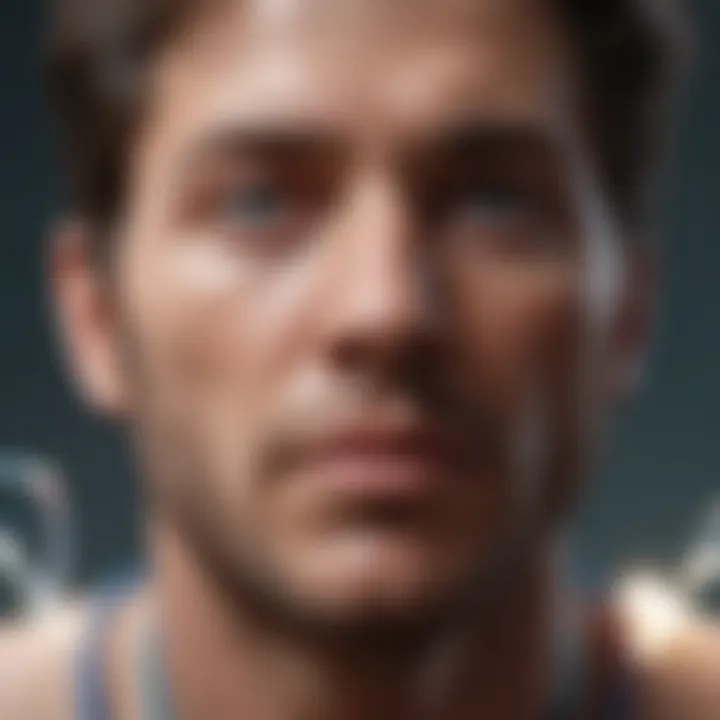
Gene Regulation
Gene regulation is like the conductor of an orchestra, guiding the timing and expression of genes in a cell. RNA translation plays a pivotal role in this symphony of genetic expression. Transcription factors bind to specific sequences in DNA, influencing which genes are turned on or off. Depending on the signals a cell receives from its environment, diverse sets of RNA molecules are synthesized.
- Control of Protein Synthesis: These RNAs then go on to dictate protein synthesis, which is essential for cellular activity. For instance, certain conditions might promote the production of stress-response proteins, while others may favor growth-promoting factors. The balance of these proteins determines cellular health and function.
- Feedback Mechanisms: Additionally, some RNAs serve as regulators themselves. MicroRNAs can bind to messenger RNAs (mRNAs), preventing them from being translated into proteins. This form of regulation provides an important feedback loop to fine-tune protein levels within the cell.
Gene regulation ensures that cells respond appropriately to internal and external stimuli, maintaining homeostasis in the organism. The nuances here are profound; consider how a neuronal cell reacts differently to stimuli compared to a liver cell. Such specificity is made possible through differentiated gene expression, initiated by various RNA molecules.
Impact on Cellular Processes
When RNA translation works seamlessly, it ensures robust cellular operation. However, when things go awry, it can lead to significant consequences. Here are a few ways that RNA impacts cellular processes:
- Cell Growth and Division: RNA translation is critical in processes such as cell growth and division. The synthesis of cyclins, proteins that regulate the cell cycle, is dependent on accurately translated RNA. Disruptions in this process can lead to uncontrolled cell proliferation and, ultimately, cancer.
- Signal Transduction: RNA also plays a key role in signal transduction pathways. When a cell receives a signal, specific RNAs can activate or inhibit responses, affecting various biological functions. For example, the expression of proteins involved in metabolism can change based on signals received from hormones, illustrating the interplay between RNA translation and cellular signaling pathways.
- Programmed Cell Death: Another critical cellular process influenced by RNA translation is apoptosis, or programmed cell death. Certain RNA transcripts are necessary for the synthesis of proteins that trigger apoptosis. Thus, adequate RNA functioning ensures that cells can effectively eliminate damaged or unnecessary cells, maintaining overall health.
Ultimately, the translation of RNA is not an isolated event but a crucial component interwoven into the fabric of cellular life. Its implications stretch across various biological pathways, influencing everything from cellular response to environmental cues to long-term stability of an organism.
In essence, the implications of RNA translation on cellular functions are profound and complex. As researchers continue to uncover new dimensions of RNA's role, the understanding that RNA is not merely a messenger but a significant player in regulating life processes becomes increasingly evident. This makes studying RNA translation not only a fascinating scientific endeavor but also paramount for advancements in medicine and biotechnology.
Evolutionary Aspects of DNA to RNA Translation
In the grand tapestry of life, the transition from DNA to RNA is not merely a mundane biochemical process; it holds profound evolutionary implications. Understanding how organisms have conserved these mechanisms offers us insights into their survival and adaptability over time. This section digs into the importance of evolution in the context of DNA to RNA translation, exploring its significance in gene regulation and the preservation of these processes across diverse species.
Conservation Across Species
The conservation of the transcription mechanism across species highlights the fundamental nature of this biological process. It suggests that all life forms share a common ancestry where the basic principles of DNA transcription have remained remarkably preserved.
- Homologous Sequences: In different species, we often find similar sequences within promoter regions that initiate transcription. This conservation implies a shared evolutionary pressure to maintain efficient gene expression mechanisms.
- Core Enzymatic Functions: The RNA polymerase enzyme, pivotal in the synthesis of RNA from a DNA template, is strikingly similar among various organisms, from simple bacteria to humans. This conservation indicates that the role of RNA polymerase is critical, likely due to its effectiveness in facilitating transcription.
- Gene Families: Many gene families have homologs across species, resulting in conserved sequences that play vital roles in organismal functions. The evolutionary maintenance of these sequences suggests that any significant change could impair functionality.
"The conservation of transcription processes underscores the efficiency of evolutionary mechanisms, where successful traits persist through natural selection."
Understanding these conservation patterns helps us make sense of why organisms react similarly to environmental pressures, even if they are miles apart on the evolutionary tree. It reflects a shared toolkit upon which life has built its diversity.
Evolution of Gene Regulation
Gene regulation has seen a fascinating evolution. Mechanisms that control gene expression have developed intricately over millions of years, adapting to meet the needs of organisms. Key points in this evolutionary development include:
- Transcription Factors: These proteins play a significant role in regulating gene expression. Over time, the divergence of transcription factors has allowed different species to adapt to varying environmental conditions while utilizing the same fundamental transcription process. This adaptability reflects an evolutionary refinement in response to both internal and external pressures.
- Enhancers and Silencers: The evolution of genetic elements such as enhancers and silencers have allowed organisms to fine-tune gene expression, optimizing it for different life stages or environmental conditions. This capability adds an extra layer of complexity to gene regulation that was not present in ancestral forms.
- Epigenetic Modifications: Such modifications, which can influence transcription without altering the DNA sequence itself, illustrate an adaptive response mechanism. The introduction of epigenetic factors has allowed for regulation that is responsive to environmental changes without necessitating genetic mutations.
As we dive deeper into the evolutionary aspects of these processes, we uncover not only the intricate nature of cellular regulation but also the resilience and adaptability of life itself. By studying the evolution from DNA to RNA, we are granted a window into both past transformations and future possibilities in genetic research and biotechnology.
Technological Applications of Transcription Mechanisms
The mechanisms of transcription are not just confined to the pages of biology textbooks or the confines of laboratory rooms. They lay the groundwork for a myriad of technological innovations and therapeutic approaches in modern science. Understanding how these processes work offers a wealth of opportunities in fields ranging from biotechnology to medicine, influencing both research and practical application.
Biotechnology Innovations
Biotechnology has taken significant leaps forward, largely due to insights gained from transcription processes. By leveraging the intricacies of RNA synthesis, researchers have developed tools that are reshaping how we approach biological problems.
- Synthetic Biology: One of the exciting branches of biotechnology, synthetic biology aims to redesign organisms by engineering genetic sequences. This is often achieved through transcriptional control mechanisms to produce beneficial biomolecules like insulin or enzymes, optimizing their production through controlled RNA expression.
- CRISPR Technology: CRISPR, widely recognized for its gene-editing capabilities, hinges on transcriptional responses to guide RNA. The ability to manipulate RNA in this context allows precise targeting of gene sequences, opening avenues for correcting genetic disorders.
- Vaccine Development: The rise of mRNA vaccines, such as those developed during the COVID-19 pandemic, is a fantastic illustration of transcriptional research in action. These vaccines employ messenger RNA to instruct cells to produce antigens, arming the immune system against diseases. This innovative approach highlights how vital transcription mechanisms are to public health initiatives.
These applications underscore the transformative impacts of transcription technologies in serving human needs and advancing research methodologies.
Important Insight: "Biotechnology innovations rooted in transcriptional mechanisms are not just tools; they are catalysts for groundbreaking scientific advancement and potential global solutions."
Gene Therapy Approaches
Another significant area benefiting from transcription mechanisms is gene therapy. Here, scientists are working to correct genetic deficiencies or diseases through direct modification of an individual’s genome. This approach often involves a deep understanding of how transcription works to ensure that the correct genes are expressed adequately.
- RNA Interference (RNAi): This technique uses RNA molecules to inhibit gene expression, often used in research and therapeutic processes. By leveraging RNAi, scientists can silence genes that might be causing diseases, providing a level of control over gene expression that was unmatched in traditional methods.
- Adeno-Associated Viruses: These are commonly used vectors in gene therapy to deliver therapeutic genes to patient cells. Understanding the transcriptional landscape helps in optimizing these vectors for effective gene delivery and expression.
- Patient-Specific Treatments: Developments in personalized medicine leverage transcriptional profiles to tailor treatments to individual patients. By examining how their unique transcriptional systems function, doctors can devise more effective, targeted therapies.
In exploring gene therapies, it's crucial to approach with a clear understanding of the ethical considerations involved. Balancing required research and development against the potential for unintended consequences remains an ongoing challenge.
Challenges in Understanding Transcription
Transcription, the process where DNA is transcribed into RNA, is a cornerstone of gene expression. However, this vital process presents multiple challenges that complicate our understanding. Getting to the heart of these challenges can greatly benefit students, researchers, and professionals alike, as they can unveil shortcomings in current methodologies and inform future studies in molecular biology.
Complexity of Regulatory Networks
The networks regulating gene transcription are intricate, resembling a web of interconnected elements. They encompass various factors, including enhancers, silencers, and transcription factors, all finely tuned to govern when, where, and how genes are activated. For instance, consider the role of enhancers, which can be located far from the gene they regulate. Their influence is not just about proximity; 3D architecture of the DNA plays a crucial role. This spatial organization complicates the study of gene regulation, as it involves understanding both linear sequences and three-dimensional arrangements within the nucleus.
Inconsistencies often arise from experiments that overlook this complexity. Researchers may focus on promoter regions while ignoring distal regulatory elements, leading to partial insights. A comprehensive grasp of these regulatory networks is essential because they can vary significantly between cell types and developmental stages. For example, what works in a muscle cell might not apply to a neuron. This variability is where research can stumble, as it’s all too easy to generalize findings from one context to another.
Experimental Limitations
While studying transcription, researchers are often up against the limits of current techniques. Many methods to analyze RNA and DNA, while effective, have their drawbacks. For instance, techniques such as ChIP-seq (Chromatin Immunoprecipitation followed by sequencing) present challenges, particularly in whole organisms. Environmental variables, differences in cellular environments, and the dynamic nature of transcription can skew results. This might lead to misinterpretations of regulatory dynamics.
Additionally, isolating RNA of the highest quality for analysis poses its own set of hurdles. RNA is notoriously unstable, and even slight mishandling can lead to degradation, impacting experimental outcomes. Hence, the challenge lies in developing methods that can preserve the integrity of RNA while also providing insights into its interaction with various regulatory elements.
"Understanding the subtleties in transcription regulation is akin to piecing together a jigsaw puzzle where the final image keeps shifting."
Ultimately, these experimental limitations, coupled with the complexity of regulatory networks, underscore the urgent need for advancements in research technologies. By tackling these challenges head-on, the scientific community may improve its understanding of transcription, leading to breakthroughs in fields such as genetics and biotechnology.
Key Takeaways
- Regulatory networks in transcription are complex and multi-faceted, often resulting in oversimplified interpretations.
- Current experimental techniques have limitations that researchers must navigate carefully to avoid misleading results.
Future Directions in RNA Research
Exploring the frontier of RNA research offers a treasure trove of possibilities that could redefine how we understand genetic expression, cellular function, and disease mechanisms. With the recent advancements in technology, the field of RNA research is set to undergo a significant transformation, potentially leading us down paths that were previously thought to be science fiction. Understanding these future directions is crucial for students, researchers, educators, and professionals who are navigating this intricate landscape.
Emerging Techniques
In recent years, several cutting-edge techniques have emerged that promise to accelerate our understanding of RNA biology. These methods are not just tools; they represent a paradigm shift in how we gather data and interpret the roles of RNA in the cellular context. Some notable techniques include:
- Single-Cell RNA Sequencing (scRNA-seq): This technology enables researchers to analyze gene expression at the single-cell level. It allows for the identification of rare cell populations, revealing how different cells respond to stimuli or disease at an unprecedented resolution.
- CRISPR-Cas Systems for RNA Targeting: Originally known for its genome-editing capabilities, the CRISPR technology is now being adapted to target and manipulate RNA molecules effectively. This evolution could open the door to new therapeutic strategies for diseases characterized by dysfunctional RNA.
- RNA-Seq for Non-Coding RNAs: Expanded RNA sequencing techniques are now focusing specifically on the vast array of non-coding RNAs, an area previously overshadowed by the attention given to protein-coding transcripts. Exploring these molecules could lead to new insights on gene regulation and cellular function.
These emerging techniques are foundational to future explorations; they’ll help create a better understanding of how RNA contributes to both normal physiology and pathophysiology.
Potential Areas of Study
As the field evolves, several potential areas for further investigation stand out. Each of these areas presents unique challenges and opportunities:
- Disease Mechanisms: Understanding the role of RNA in various diseases, including cancer and neurodegenerative disorders, can pave the way for novel treatment approaches. Research will focus on how aberrant RNA expression correlates with disease progression.
- Therapeutic RNA Manipulation: The use of RNA as a therapeutic target or even a therapeutic agent itself is gaining traction. Further work is needed to explore RNA interference, aptamers, and other RNA-based therapies to treat diseases at the molecular level.
- Evolutionary Perspectives: Delving deeper into how RNA functions across different species could reveal insights into evolutionary biology. The study of conserved RNA elements may uncover fundamental biological principles that transcend species boundaries.
Understanding RNA dynamics is not just a scientific endeavor; it’s a cornerstone of modern biology that can drive innovations in healthcare and biotechnology.