Mechanisms of Membrane Protein Transport and Their Impact
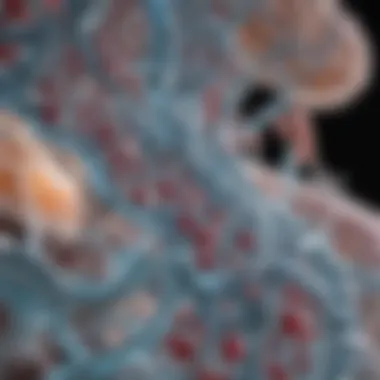
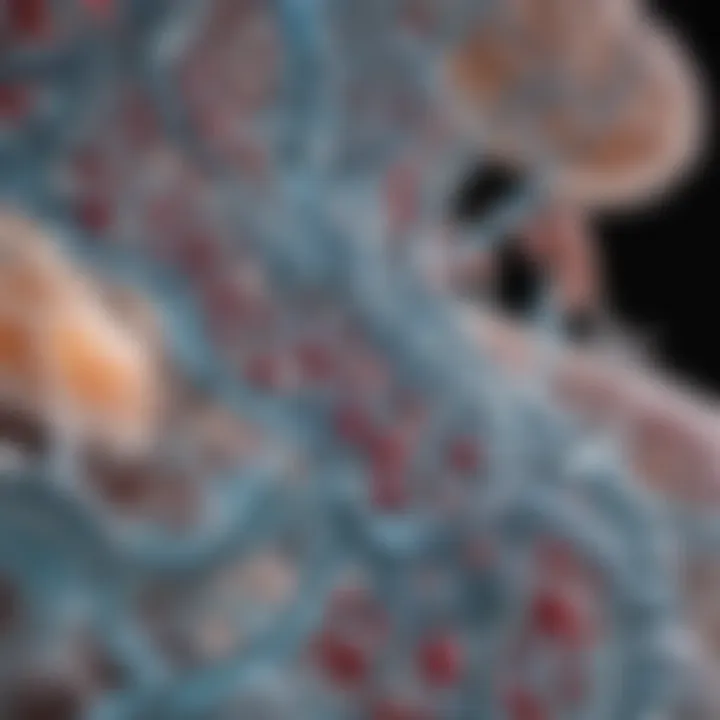
Intro
Understanding how proteins navigate the complex landscape of cellular membranes is a fascinating and essential area of study in biology. Membrane proteins are not merely passive conduits; they engage in myriad biological processes, influencing everything from nutrient uptake to signal transduction. Their movement across membranes often involves sophisticated mechanisms, which can vary significantly across different contexts.
It’s not just about getting from point A to point B; it’s about the nuances that dictate how they function within their cellular environments.
In this exploration of membrane protein transport, we will cover how these proteins are categorized, the mechanisms that facilitate their movement, and the underlying factors that can impact their behavior. Additionally, we’ll touch upon the implications of these transport mechanisms for health and disease, highlighting why this subject holds paramount importance in the realms of cellular biology and therapeutic innovation.
Let's dive into the specific methods that researchers use to study these intricate transport systems.
Prelude to Membrane Proteins
The realm of membrane proteins is a vast and intricate one, playing pivotal roles in virtually every cellular process. At the heart of cellular function, these proteins not only ensure the structural integrity of cell membranes but also act as gatekeepers, selectively allowing substances to pass in and out. Understanding membrane proteins is crucial because anything affecting their function can lead to dire biological consequences.
Definition and Importance
Membrane proteins are integral components of living cells, nestled within the lipid bilayer of cellular membranes. They can be broadly categorized into two types: integral and peripheral proteins. The reason this classification holds significance is that it allows researchers to understand the distinct functions they serve.
Integral proteins are often involved in transport processes, acting as channels or carriers for various molecules, including ions and nutrients. In contrast, peripheral proteins typically play a role in signaling, adhering to the surface of membranes and interacting with other protein structures.
The significance of membrane proteins also lies in their involvement in numerous biological functions. For instance, they are crucial for processes like signal transduction, immune response, and nutrient uptake. Given their diverse roles, the dysfunction of these proteins can be linked to a range of diseases, including cancer, diabetes, and cardiovascular disorders. This connection underscores the necessity for a detailed examination of their mechanisms and implications, which this article aims to cover.
Types of Membrane Proteins
Integral Membrane Proteins
Integral membrane proteins are embedded within the lipid bilayer and are often traversing through it. This unique positioning allows them to function as gateways for the transport of molecules across the membrane. Since they span the membrane, they exhibit amphipathic characteristics; having both hydrophilic and hydrophobic domains. This feature is essential as it facilitates interactions with both the aqueous environment and the lipid core of the membrane.
Their importance can't be understated; these proteins mediate many transport processes essential for cellular function. For example, ion channels and transporters are key for maintaining the ion balance inside a cell. The mechanisms these proteins use can vary widely, making their study fascinating. However, a limitation exists as well: since they are challenging to isolate and study due to their hydrophobic nature, research in this area often relies on technological advancements in imaging and purification strategies.
Peripheral Membrane Proteins
On the other side of the spectrum, peripheral membrane proteins don't penetrate the lipid bilayer. Instead, they associate loosely with the membrane's exterior or interior surfaces, relying on weaker interactions such as hydrogen bonds or ionic bonds. This distinctive feature makes peripheral proteins particularly adaptable, allowing them to rapidly dissociate and reassociate with other proteins or lipids in response to cellular signals.
Considered vital for cellular communication, these proteins play roles in signaling pathways and cytoskeletal interactions. Notably, they often serve as biochemical markers, indicating particular physiological states or functions in a cell. However, their loosely attached nature can also lead to challenges regarding stability and specificity, making their study complex yet enriching.
Through exploring both types of membrane proteins, this article will delve into their mechanisms, roles, and implications, providing insights that could aid in future research efforts across various biological fields.
Mechanisms of Membrane Protein Transport
Understanding the mechanisms of membrane protein transport is like peeling back an onion; each layer reveals the critical roles these processes play in cellular function. The movement of proteins across cellular membranes isn't just a routine process; it's fundamental to maintaining the delicate balance of cellular environments. These mechanisms are essential for numerous physiological functions, including nutrient uptake, waste removal, and signal transduction. When broken down into their components, we see how transport can be categorized into passive and active transport, each with its own unique features and implications.
Passive Transport
Passive transport encompasses the movements of substances across membranes without the expenditure of energy. Essentially, proteins utilize existing concentration gradients to facilitate movement, making it a naturally efficient process. This type of transport is particularly important because it enables cells to maintain homeostasis, manage energy resources effectively, and respond swiftly to changing environmental conditions.
Facilitated Diffusion
Facilitated diffusion stands at the forefront of passive transport mechanisms. This method is characterized by the assistance provided by transport proteins embedded within the cell membrane, which help hydrophilic substances navigate the lipid bilayer. One key aspect of facilitated diffusion is its selectivity; different transport proteins cater to specific molecules. For instance, glucose transporter proteins ensure that glucose molecules can enter the cell as their concentration outside is higher than inside.
"Facilitated diffusion is like a doorman ensuring only the right guests enter the party."
Despite its advantages, facilitated diffusion does have limitations. For one, it can only move substances down their concentration gradient, meaning it cannot transport molecules against the established gradient. This can be a significant drawback when rapid uptake of nutrients is necessary in environments where they are scarce.
Osmosis
Osmosis, while often associated with water movement, is a crucial aspect of passive transport that warrants deeper discussion. This process only allows water to move across a selectively permeable membrane. One key characteristic of osmosis is its reliance on solute concentration; water moves toward areas of lower water concentration (higher solute concentration) until equilibrium is reached. The uniqueness of osmosis lies in its ability to regulate the cell's internal environment by balancing solute concentrations, making it vital for cellular survival and function.
The advantages of osmosis are clear, especially in the dynamic environments cells face. However, there can be disadvantages as well. An excessive influx of water can lead to cell lysis, highlighting the need for cells to regulate osmotic pressure diligently.
Active Transport
Active transport is quite different from its passive counterpart. This mechanism requires energy, often from ATP, to move substances against their concentration gradients. Active transport plays a pivotal role in maintaining unique intracellular environments and ensuring cells can gather necessary ions and molecules even in unfriendly conditions.
Primary Active Transport
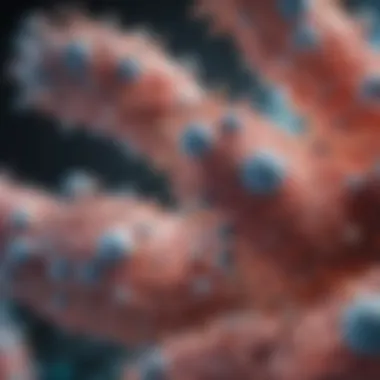
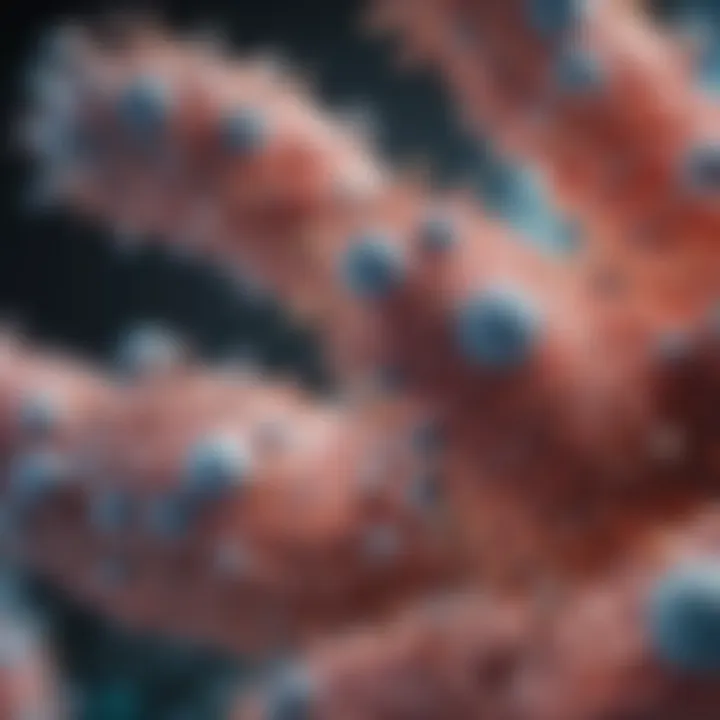
The most prominent example of primary active transport is the sodium-potassium pump. This system uses ATP to exchange sodium ions out of the cell and potassium ions in, crucial for maintaining resting membrane potential and cellular homeostasis. The high energy requirement of this mechanism is both its strength and weakness—while it allows cells to establish steep ion gradients, excessive energy usage can be a strain under certain conditions.
Secondary Active Transport
Secondary active transport, which can also be termed as co-transport, relies on the gradients established by primary active transport. Instead of directly using ATP, this mechanism harnesses the ion concentrations created to facilitate the transport of other substances. The hallmark of secondary active transport is its ability to contribute to cellular processes efficiently, as many nutrients can be brought into the cell alongside sodium ions due to the sodium gradient created by primary active transport methods.
Both forms of active transport are essential, providing versatility and adaptability for cells as they respond to an ever-changing environment. However, they also require careful balance since over-reliance on these mechanisms can trigger resource depletion or cellular stress.
Understanding these transport mechanisms is vital, as they not only shape cellular function but also have ramifications in health and disease. As we explore further, we will look into the proteins responsible for these transports, the factors that influence their efficacy, and how these systems relate to our well-being.
Transport Proteins: Key Players
When it comes to the fascinating world of membrane proteins, transport proteins are essential players in the orchestration of cellular function. These proteins facilitate the movement of substances across the otherwise impermeable lipid bilayer of the cell membrane. Understanding their role is crucial for comprehending how cells maintain their internal environments and respond to external signals.
Importance of Transport Proteins
Transport proteins can be broadly categorized into two main types: channel proteins and carrier proteins. Each type serves specific functions that are vital for the survival of cells and, by extension, the organism.
- Channel proteins act as gateways, allowing specific molecules to flow in and out of the cell freely. This process is usually passive and depends on concentration gradients, meaning substances move from areas of high concentration to low concentration.
- Carrier proteins, on the other hand, often require energy input to transport molecules against their concentration gradient. This characteristic is extremely important for the uptake of nutrients and the expulsion of waste products, ultimately contributing to the cell's homeostasis.
Here are some of the key benefits and considerations associated with transport proteins:
- Regulation of Intra- and Extracellular Conditions: Transport proteins maintain the critical balance of ions, nutrients, and waste products, which is crucial for cellular operations.
- Signal Transduction: Some transport proteins are involved in signaling pathways, which are paramount for a cell's response to its environment.
- Drug Targeting: Many transport proteins are involved in drug absorption and distribution, making them attractive targets for pharmacological interventions.
As we delve deeper into the specifics, it's vital to recognize that dysfunction in these proteins can lead to various disorders, underscoring their importance not only in physiology but also in understanding pathology.
"Transport proteins don't just help molecules cross membranes—they help define cellular destiny."
Channel Proteins
Channel proteins are like turnstiles at a subway station. They selectively enable specific ions and molecules to pass through the membrane with ease, often driven by passive transport mechanisms. Their structure typically includes a hydrophilic passage that allows polar molecules or ions to move in response to concentration gradients.
A classic example is the voltage-gated sodium channels, which play a pivotal role in neuronal signaling. When the membrane potential reaches a certain threshold, these channels open up, allowing sodium ions to rush in. This influx of sodium ions is what triggers the action potential, a fundamental process in nerve impulse transmission.
Another notable mention is aquaporins, which allow for rapid water transport across the membrane, critical for osmoregulation in cells.
Carrier Proteins
Carrier proteins can be envisioned as bouncers at a club, deciding who gets in and how. These proteins undergo conformational changes to transport substances across the membrane. In many cases, the transport can be active, requiring energy in the form of ATP to move against a gradient.
Take, for example, the glucose transporter (GLUT). This protein facilitates the uptake of glucose into the cell, a process that is essential for energy production. The ability of carrier proteins to switch between different shapes—open inside the cell, then outside after the molecule binds—enables them to perform this complex task efficiently.
In essence, channel and carrier proteins represent two sides of the same coin, each playing irreplaceable roles in ensuring the cellular environment is optimal for life's processes.
Factors Influencing Membrane Protein Transport
Understanding factors that influence membrane protein transport is vital. It allows researchers to grasp how proteins operate within the cellular milieu and their implications for health and disease. The efficiency of transport mechanisms can be modified by various elements, such as concentration gradients and membrane potential. Let's explore these in detail.
Concentration Gradients
Concentration gradients act as the fundamental driving force behind many transport processes. The difference in concentration of a particular substance across a membrane creates a gradient that proteins and other molecules try to balance.
- In passive transport, molecules naturally move from areas of high concentration to low concentration, seeking equilibrium. For instance, in the case of oxygen diffusing into a cell, it travels from an area of high oxygen concentration outside the cell to lower concentration inside the cell.
- The gradient doesn't just apply to small molecules. Ions and larger molecules also rely on these gradients. For example, the flow of sodium and potassium across nerve cell membranes underlies the action potentials crucial for nerve signaling.
It's important to note that concentration gradients do more than just passively facilitate movement; they also influence how effective carrier and channel proteins function. If the gradient is steep, transport can occur swiftly, but as equilibrium approaches, transport rates may diminish.
"Membrane transport is like a dance—perfect harmony between the dancers (molecules) and the song (gradient) they follow."
Membrane Potential
Membrane potential refers to the voltage difference across a membrane. This potential difference plays a significant role in the transport of charged particles, especially ions.
- The resting membrane potential is crucial for how neurons and muscle cells activate. For example, while at rest, neurons maintain a negative internal charge, attracting positively charged sodium ions. When the neuron is stimulated, the influx of these ions leads to depolarization, paving the way for action potentials.
- Alterations in membrane potential can dramatically change the state of transport proteins. If depolarization occurs, it can increase the permeability of the membrane to certain ions or molecules, allowing for a rapid change in concentration gradients across the membrane.
Examples of Membrane Protein Transport Systems
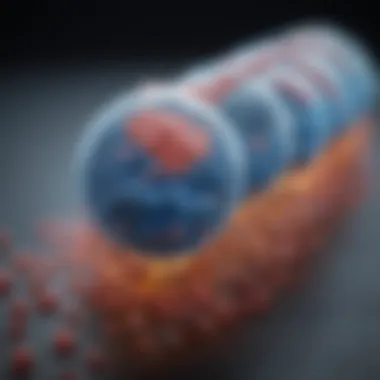
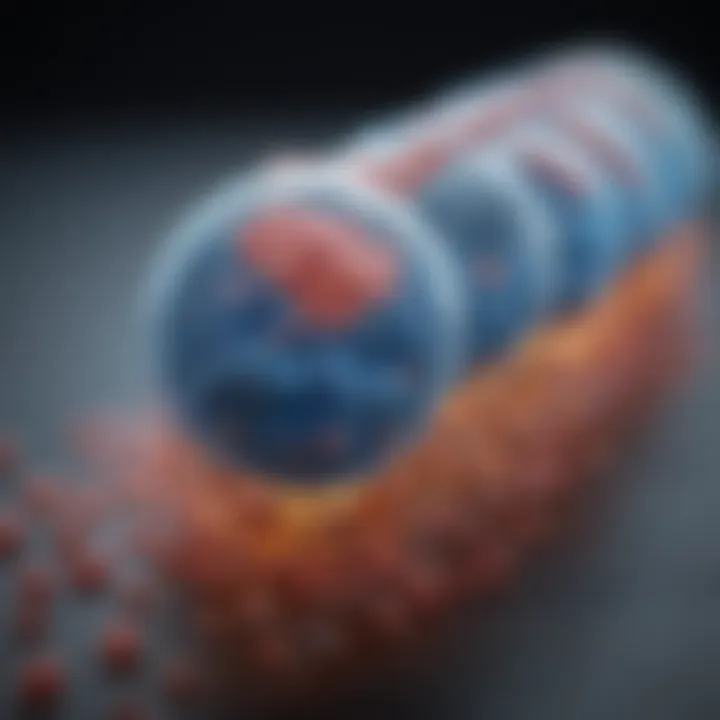
Membrane proteins are the gatekeepers of cellular transport, playing pivotal roles in maintaining homeostasis and facilitating essential biochemical processes. Understanding specific examples of membrane protein transport systems allows us to appreciate how cells regulate their internal environment. These systems not only illustrate the mechanisms at work but also provide insights into how disruptions can lead to health issues.
Sodium-Potassium Pump
The sodium-potassium pump, often referred to as the Na+/K+ ATPase, is a quintessential example of active transport. Each cycle of this pump moves three sodium ions out of the cell while bringing two potassium ions in. This activity is crucial for maintaining a negative charge inside the cell, integral for processes like nerve impulse transmission and muscle contraction.
The pump functions using ATP, which provides the necessary energy to move ions against their concentration gradients. The significance of this mechanism cannot be overstated; without it, our cells would quickly lose their ability to function. The Na+/K+ ATPase contributes to cellular volume regulation and sets the stage for other transport mechanisms.
A well-functioning sodium-potassium pump ensures that our cells are ready for the physiological demands placed upon them, stabilizing the excitability of neurons and maintaining muscle integrity.
While the sodium-potassium pump is essential for health, mutations or malfunctions can lead to diseases such as cardiac failure and hypertension. This emphasizes the need to study not just how the pump works but how it can be targeted in therapy for various conditions.
Glucose Transporter
Another critical example is the glucose transporter, specifically SGLT1 (Sodium-Glucose Transporter 1) and GLUT1 (Glucose Transporter 1). These transport proteins are integral to glucose uptake in the intestines and other tissues, facilitating energy production in cells. They showcase both passive and active transport mechanisms.
SGLT1 utilizes the sodium gradient established by the sodium-potassium pump, coupling the transport of sodium ions with glucose. This starch and sugar duo exemplifies how different transporters can work together to ensure that cells obtain necessary nutrients efficiently. On the other hand, GLUT1 operates simply on the principle of facilitated diffusion, allowing glucose to flow down its concentration gradient into cells, particularly those that require high glucose levels like erythrocytes.
Understanding these transporters is pivotal, especially considering their implications in diseases such as diabetes. In diabetic patients, the functionality of glucose transporters can be severely impaired, leading to elevated blood sugar levels and associated complications.
The effectiveness of these transport systems opens avenues for pharmacological intervention which could enhance glucose uptake in insulin-resistant individuals.
Role of Membrane Protein Transport in Health
The transport of membrane proteins holds significant ramifications for our health, intertwining with various physiological processes that contribute to homeostasis and the overall well-being of organisms. These proteins, embedded within the lipid bilayer of cell membranes, are paramount for controlling the internal environment of cells and the communication between different tissues.
Cellular processes such as nutrient uptake, waste removal, and signaling pathways hinge on the functioning of these transport proteins. Any defect or dysfunction in these transport mechanisms can trigger a cascade of health issues, highlighting the critical nature of membrane protein transport in health.
"The complexity of membrane protein transport is an ever-openeing window into understanding human biology."
Cellular Homeostasis
Maintaining cellular homeostasis is fundamental for life. Membrane proteins act like gatekeepers, regulating what enters and exits a cell. Ion channels, for example, allow the selective passage of ions, balancing charges within the cell. Likewise, nutrient transporters like glucose transporters are crucial in providing cells with glucose, which is a key source of energy.
The importance of these proteins becomes evident when considering conditions like diabetes. In this case, the function of glucose transporters is compromised, leading to elevated glucose levels in the bloodstream. This situation illustrates how disrupting the transport system can lead to significant metabolic disorders.
Furthermore, the process of osmosis, driven by the movement of water through aquaporins—specialized channel proteins—also showcases how membrane proteins maintain volume and pressure within cells, ensuring they function efficiently.
Impact on Drug Delivery
The implications of membrane protein transport extend beyond normal physiological function and touch on drug delivery, an area that has garnered a great deal of attention in recent years. Achieving effective therapeutic outcomes often relies on understanding how drugs interact with transport proteins. Many medications are designed to target specific transporters; for instance, many antidepressants and antihypertensives use mechanisms that involve these proteins to modulate their effects.
A practical example can be seen with the cancer treatment known as Imatinib (Gleevec), which specifically targets the BCR-ABL protein in leukemia cells. Its effectiveness hinges on the ability of the drug to enter the cells via transport mechanisms. This targeting not only enhances the efficacy of the drug but also minimizes side effects by ensuring that the treatment affects only the intended tissues.
In summary, the role of membrane protein transport is multifaceted, directly influencing cellular homeostasis and impacting modern therapeutic strategies. Understanding these dynamics helps pave the way for innovative approaches in treating various health conditions, thereby underscoring the critical link between membrane protein transport and health.
Dysfunction of Membrane Protein Transport in Disease
Understanding the dysfunction of membrane protein transport is pivotal in comprehending how various diseases manifest and progress. Membrane proteins perform essential functions, and their proper transport is integral to cellular homeostasis and communication. When these transport mechanisms falter, a cascade of complications can arise, affecting not just individual cells, but the entire organism. Hence, exploring this topic unveils vital implications for diagnosing and treating diseases, ultimately enhancing our grasp of biological processes.
Genetic Disorders
Genetic disorders represent a category where disruptions in membrane protein transport can lead to profound health implications. Mutations in genes encoding membrane proteins often result in dysfunctional transport processes. For instance, cystic fibrosis is a genetic condition stemming from mutations in the CFTR gene, which encodes a chloride channel. The dysfunctional transport of chloride ions results in thick mucus buildup in organs, particularly the lungs. This not only impedes respiratory function but also sets the stage for frequent infections.
Another example is the Niemann-Pick disease, which arises from a defect in lipid transport. The lack of proper transport leads to lipid accumulation in cells, which can damage various organs and affect neurological function.
Here are key points to consider regarding membrane transport dysfunction in genetic disorders:
- Inheritance Patterns: Understanding how these disorders are passed down helps in identifying at-risk populations.
- Clinical Manifestations: Each disorder may present a unique set of symptoms based on which transport protein is affected and its functional role.
- Research and Treatments: Advances in gene therapy indicate potential pathways for corrective measures targeting these transport issues.
It’s noteworthy how genetic disorders exemplify that even small alterations at the molecular level can translate into significant health issues, emphasizing the critical nature of proper membrane transport.
Metabolic Disorders
Metabolic disorders also showcase the importance of membrane protein transport in overall health. These conditions typically arise when metabolic processes are disrupted, often due to dysfunctional transport proteins which can impact nutrient uptake, waste removal, and energy production. Consider diabetes mellitus, a disease where insulin transport is crucial. In type 1 diabetes, the body's inability to produce insulin affects glucose transport into cells, leading to elevated blood sugar levels.
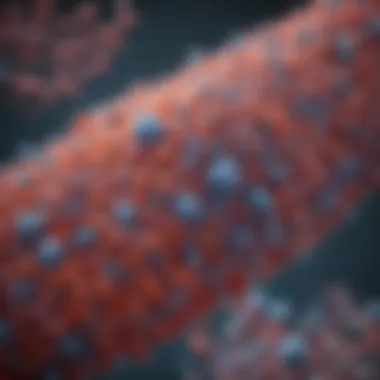
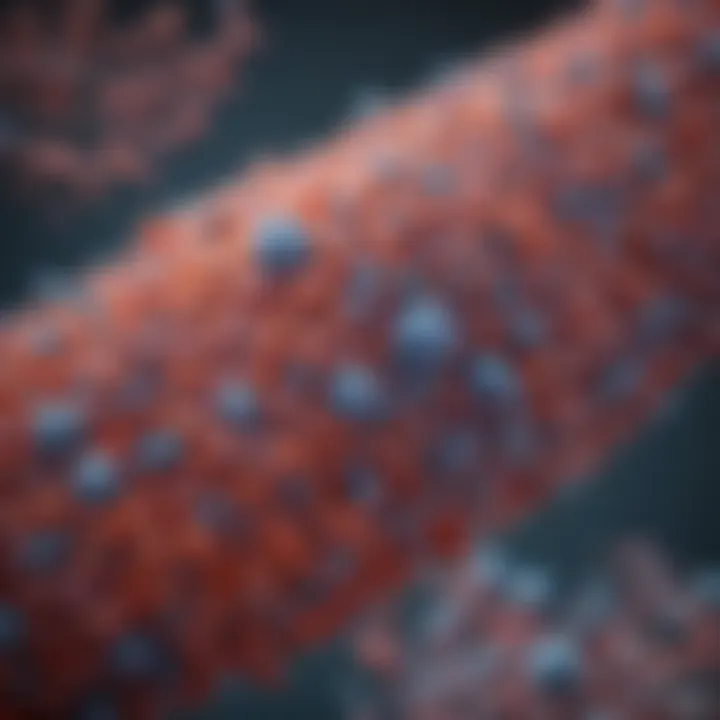
In cases of inherited metabolic disorders such as phenylketonuria (PKU), the impaired transport of certain amino acids can lead to toxic build-up and neurological damage if not managed through dietary restrictions.
- Sugar Transport: Disorders in glucose transport proteins can cause serious issues in the management of糖尿病.
- Lipid Metabolism: Disorders like familial hypercholesterolemia arise due to defective LDL receptors, resulting in excessive cholesterol levels in the bloodstream.
To sum up, the interplay between membrane protein transport and metabolic processes elucidates how dysfunction can derail cellular activities.
"The intricacies of membrane transport are the keys to unlocking numerous therapeutic strategies."
As research continues to progress, a deeper understanding of these transport mechanisms in diseases is paramount for developing effective treatments and prevention strategies.
Research Trends in Membrane Protein Transport
The field of membrane protein transport is undergoing a significant shift, driven by advances in technology and collaborative efforts across scientific disciplines. Understanding the latest trends in this area is crucial, especially considering the role membrane proteins play in health and disease. Researchers and practitioners must keep their fingers on the pulse of these developments to grasp the implications they hold for therapeutic strategies and cellular function.
Technological Advances
The evolution of research methodologies in membrane protein transport is nothing short of revolutionary. Techniques that were once thought to be the stuff of dreams are now reality. One notable advance is the use of cryo-electron microscopy, which allows scientists to visualize membrane proteins in their near-native state. This method provides a clearer picture of protein structures and their interactions within the membrane, much like taking a detailed snapshot of a bustling city street at midnight.
Another important tool in the toolkit is single-molecule fluorescence microscopy. This allows researchers to observe the behavior of individual proteins in real-time, shedding light on dynamic processes such as channel opening and closing. The level of detail available through these techniques raises the bar significantly for our understanding of transport mechanisms.
Additionally, protein engineering has taken center stage. With the rise of CRISPR technology, scientists can now modify proteins at a genetic level, creating variants that can offer new insights into functionality and transport pathways. This is akin to being able to tweak parts of an engine and watch how it performs differently on the road.
Interdisciplinary Approaches
In today's complex biological landscape, no single discipline can claim to have all the answers. Researchers are increasingly adopting interdisciplinary approaches that combine elements from biochemistry, physics, and computational biology. This collaboration not only enriches the data pool but also fosters innovative thinking.
For instance, data scientists are utilizing machine learning algorithms to predict how changes in a protein’s structure could affect its transport efficiency. Such predictive modeling can expedite the identification of potential drug candidates or even new therapeutic methods for diseases caused by dysfunctional transport proteins.
Collaboration among researchers from different backgrounds is yielding promising strategies to tackle challenges like drug resistance, which is often linked to membrane transport dysfunction. A biochemist might work hand-in-hand with a computational modeler to develop a comprehensive approach tailored to specific diseases, much like two chefs combining unique spices to create an exquisite dish.
Recent studies show that interdisciplinary projects can enhance innovation and improve the speed of scientific discovery, propelling membrane protein transport research into new territories.
As we glance into the future, it’s clear that trends in technology and collaboration are not just enhancing our understanding of membrane protein transport, but they’re also paving the way for groundbreaking therapeutic interventions. Keeping an eye on these developments is paramount for researchers and professionals engaged in biomedicine and related fields.
Future Directions in Membrane Protein Research
As we look forward, pinpointing the future directions in membrane protein research remains crucial. Understanding these proteins at a deeper level can provide revolutionary chances for medical advancements and innovations in biotechnology. With the complexity of cellular environments, membrane proteins often play critical roles beyond mere transport, affecting cellular signaling and interaction with various molecules. Knowing how to manipulate or understand these processes can lead to major breakthroughs in health and disease management.
Potential Therapeutic Targets
Identifying membrane proteins as therapeutic targets has become a primary focus in modern biomedical research. Many diseases, from diabetes to various cancers, have underlying issues in membrane protein functions. This highlights why evaluating these proteins offers a path to development of new treatment options. For instance, drugs that inhibit certain transport proteins can effectively alter the concentration of essential ions or molecules in affected tissues.
Potential therapeutic strategies include:
- Targeted Drug Delivery: Utilizing carrier proteins to ferry medication directly into diseased cells.
- Gene Therapy: Modulating gene expression related to dysfunctional membrane proteins to restore normal function.
- Small Molecule Inhibitors: Developing small molecules that can either activate or deactivate specific transport mechanisms for more effective treatment.
These methods underscore the significance of thorough research into the mechanisms of membrane transport. By exploring these pathways, researchers can unveil previously undiscovered targets that could significantly enhance current therapeutics.
Innovations in Research Methodologies
Progress in the field often coincides with technological advancements. New techniques are shaping how scientists study membrane proteins, allowing for more detailed and intricate examinations than ever before. Innovations include:
- Cryo-Electron Microscopy: This cutting-edge method permits imaging of proteins in their native state, providing insights into their structures and functions under physiological conditions.
- Single-Molecule Approaches: These methods allow researchers to observe membrane protein behavior in real-time, understanding how they function at an individual level without averaging out their responses.
- High-Throughput Screening: Utilizing robotic systems to rapidly test numerous compounds against membrane proteins, accelerating the identification of potential therapeutic agents.
These evolving methodologies reveal not just the form and function of membrane proteins, but also facilitate collaboration across disciplines such as biophysics and computational biology. As research expands, the integration of diverse scientific approaches will enhance our understanding and treatment options in diseases related to membrane proteins.
"The exploration of membrane proteins paves the way for therapeutic innovations that can redefine treatment strategies in various medical fields."
The ongoing innovation in research methodologies complements the identification of potential therapeutic targets, driving future projects that could yield significant scientific contributions ultimately addressing pressing health concerns. The interplay of technology and biology holds great promise for understanding and harnessing the power of membrane protein transport.
Closure
Understanding the transport of membrane proteins is paramount for grasping cellular processes and their implications in health and disease. Those proteins play a critical role in controlling what enters and exits cells, maintaining cellular integrity and homeostasis. With biological systems growing increasingly complex, the study of membrane protein transport offers profound implications for medicine, particularly in the realm of targeted therapies and disease treatment.
Summary of Key Points
- Membrane Proteins: They are essential for various physiological processes, influencing how substances move across cellular barriers.
- Transport Mechanisms: The article explores both passive and active transport, detailing how proteins facilitate the movement of molecules in different contexts.
- Health Implications: A deep understanding of transport mechanisms can lead to advancements in drug delivery systems and therapies, directly impacting patient care.
- Dysfunctions and Diseases: Genetic and metabolic disorders often manifest through impaired transport mechanisms, highlighting the need for ongoing research in this field.
- Future Directions: Innovations and interdisciplinary approaches are crucial for uncovering novel therapeutic targets and enhancing our research methodologies in membrane biology.
Final Thoughts on Membrane Protein Transport
Membrane protein transport is not just a fundamental biological concept but a cornerstone in the pursuit of medical advancements. As we expand our understanding of how these proteins operate, we move closer to unlocking critical insights into conditions that affect millions. The ongoing research within this sphere is crucial, marking the path toward innovative treatments and efficient drug delivery systems. Collaborations across disciplines will be essential as we delve deeper into the intricacies of membrane protein functions. The future of therapeutic development may very well hinge on insights gained from elucidating the mechanisms governing membrane protein transport.