Converting DNA to RNA Sequence: Comprehensive Guide
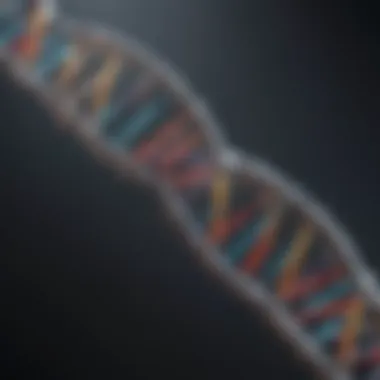
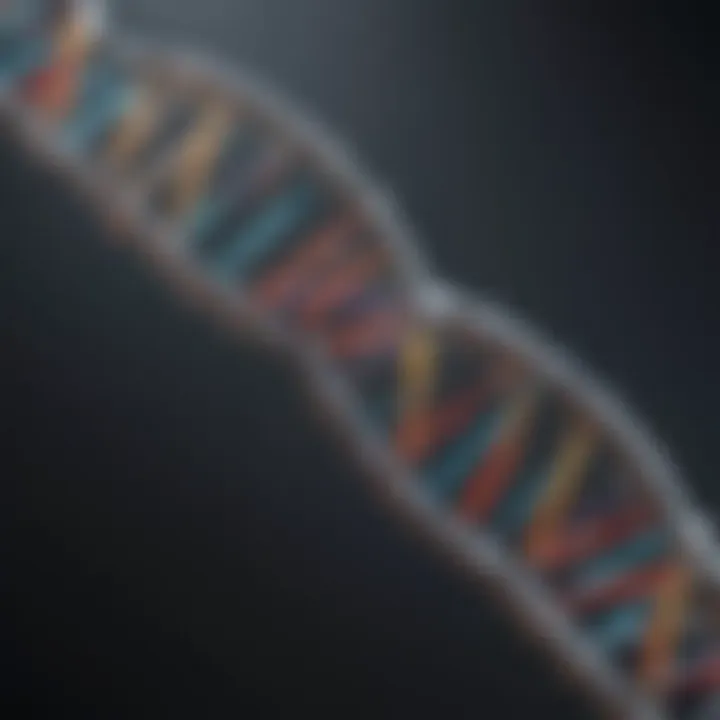
Intro
The conversion of DNA to RNA is a crucial process in the field of molecular biology. This transformation, known as transcription, serves as the bridge between genetic information encoded within DNA and the functional proteins produced through translation. Understanding how this process works is essential for students, researchers, and educators in the scientific community. This guide aims to dissect the mechanisms involved, describe the roles of various enzymes, and discuss the broader implications for research and biotechnology.
Methodology
Overview of research methods used
The study of the transcription process employs various methods that span both in vitro and in vivo scenarios. In vitro techniques often involve isolated DNA and RNA polymerase enzymes, enabling researchers to analyze transcription under controlled conditions. Whereas in vivo approaches utilize living cells, providing a richer context for understanding the dynamic environment in which transcription occurs. Common methodologies include:
- Polymerase Chain Reaction (PCR): Used for amplifying specific DNA sequences.
- RNA Sequencing (RNA-Seq): A technique that reads RNA sequences to reveal gene expression patterns.
- Microarray Analysis: A method for measuring gene expression on a large scale.
Data collection techniques
For gathering data on the transcription process, various techniques are implemented. These methods range from qualitative analyses to quantitative measurements. Here are a few notable techniques:
- Chromatin Immunoprecipitation (ChIP): This method is invaluable for studying the interaction between DNA and proteins.
- Northern Blotting: Used to detect specific RNA sequences within a complex mixture.
- Fluorescent In Situ Hybridization (FISH): Allows for visualizing the location of specific RNA sequences in cells.
Each of these methods contributes to a comprehensive understanding of how DNA is transcribed into RNA, providing insights into both the process and its regulatory mechanisms.
Future Directions
Upcoming trends in research
The continual evolution of technology promises to unlock new avenues for studying transcription. Single-cell RNA sequencing is emerging as a significant trend, allowing researchers to explore gene expression at an unprecedented resolution. Additionally, advancements in CRISPR technology will enhance our ability to manipulate transcriptional processes effectively, leading to innovative biotechnological applications.
Areas requiring further investigation
Despite significant advancements, several areas in transcription research need further exploration. The role of non-coding RNAs, for instance, remains poorly understood, encompassing a wide range of potential functions in gene regulation. Another critical area is the study of transcription factors and their interactions, which play a vital role in determining cell function and identity.
"Understanding the nuances of transcription not only informs fundamental science but also has practical implications in biotechnology and medicine."
In summary, this guide serves as a comprehensive overview of converting DNA to RNA sequences, shedding light on methodologies, data collection techniques, and directions for future research.
Prelims to DNA and RNA
Understanding the basics of DNA and RNA is a critical foundation for exploring the processes that lead to gene expression. The conversion from DNA to RNA, known as transcription, plays a significant role in the functioning of all living organisms. This article will examine how DNA and RNA differ in structure and function, emphasizing their roles in genetic information transfer and expression.
Defining DNA and RNA
DNA, or deoxyribonucleic acid, is the molecule that carries the genetic instructions for life. It is comprised of two long strands forming a double helix, which encodes the information necessary for the development, functioning, and reproduction of organisms. RNA, or ribonucleic acid, is a single-stranded molecule that acts as a messenger, transferring the genetic information from DNA to the sites of protein synthesis.
The main types of RNA include messenger RNA (mRNA), transfer RNA (tRNA), and ribosomal RNA (rRNA), each playing distinct roles in the biological processes within cells.
The Structure of DNA
The structure of DNA is vital to its role in genetics. It consists of a backbone made of sugar and phosphate groups, with nitrogenous bases (adenine, thymine, cytosine, and guanine) attached to the sugar. The specific pairing of these bases—adenine with thymine and cytosine with guanine—allows DNA to exist as a double helix. This configuration not only protects the genetic material but also provides a stable platform for replication and repair.
Furthermore, the arrangement of genes along the DNA molecule can directly influence how and when a gene is expressed, which underscores its importance in regulating biological functions.
The Structure of RNA
In contrast to DNA, RNA has a simpler structure. It is composed of a single strand made up of ribose sugar, phosphate, and nitrogenous bases (adenine, uracil, cytosine, and guanine). Uracil replaces thymine found in DNA. This alteration in structure affects how RNA functions within the cell.
The single-stranded nature of RNA allows it to fold into various shapes, which is critical for its role in protein synthesis. For example, mRNA carries the genetic code from DNA to ribosomes, while tRNA assists in translating that code into the amino acid sequence of proteins.
Understanding the structural differences between RNA and DNA is crucial for grasping how information is stored, transmitted, and utilized within biological systems.
The Importance of Transcription
Transcription is a critical process in molecular biology where DNA is converted into RNA. This process serves as a bridge between genetic information and the active expression of genes. Understanding transcription is fundamental because it lays the groundwork for how organisms function at a cellular level.
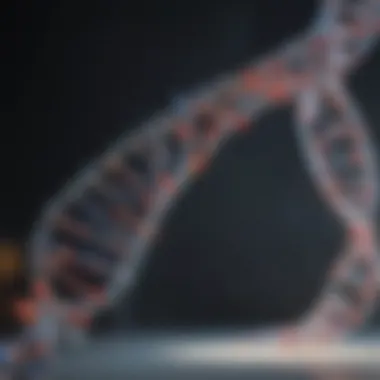
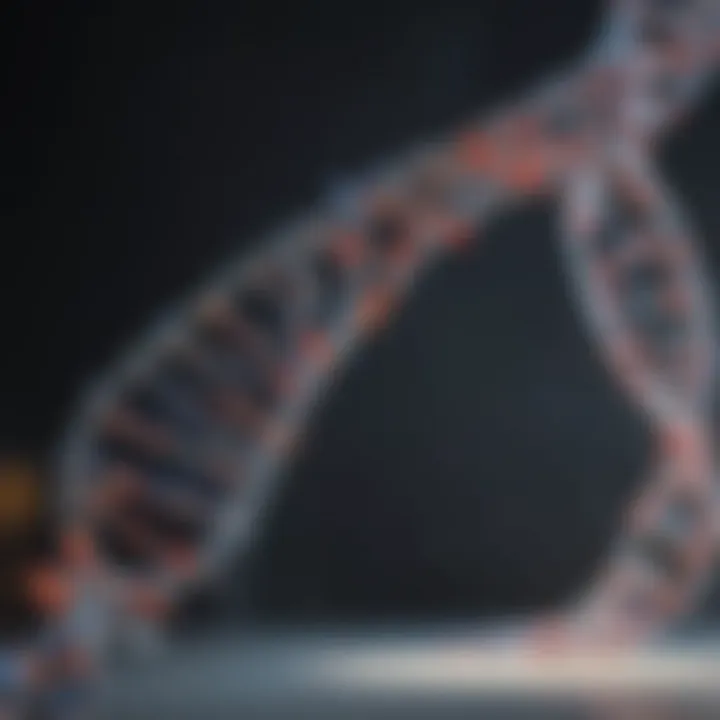
Role in Gene Expression:
Transcription is the first stage of gene expression. It allows the information written in DNA to be transformed into a usable form: RNA. This RNA can then be translated into proteins, which are essential for countless cellular functions. Without transcription, cells would lack the necessary RNA to create proteins, ultimately leading to dysfunction.
Regulation of Cellular Activities:
Transcription also plays a key role in regulating cellular activities. The timing and quantity of transcription of specific genes can determine how cells respond to internal and external signals. This regulation is crucial for processes such as development, cellular response to stimuli, and homeostasis. Moreover, misregulation of transcription is often implicated in diseases like cancer.
Implication for Biotechnology and Medicine:
In biotechnology, understanding transcription can lead to innovations in genetic engineering, synthetic biology, and therapeutics. For example, researchers can manipulate transcription factors to influence which genes are expressed in genetically modified organisms. This has applications in agriculture as well as in developing treatments for genetic disorders.
To summarize, transcription is not just a biochemical step; it is essential for life's machinery. Its significance spans various fields, affecting everything from basic biology to advanced applications in biotechnology and medicine.
Understanding Genetic Expression
Genetic expression refers to the process by which information from a gene is utilized to synthesize functional gene products, typically proteins. This process involves two major steps: transcription and translation.
- Transcription: The DNA sequence of a gene is transcribed to produce messenger RNA (mRNA). This step happens within the cell nucleus.
- Translation: The mRNA is translated into a specific protein at the ribosome, guided by RNA sequences known as codons.
Key Elements of Genetic Expression:
- Gene Regulation: Factors that control gene expression play a significant role in determining which proteins are produced and in what quantities. This can depend on environmental factors, lifestyle, and developmental stage.
- Chromatin Structure: The organization of DNA into chromatin affects transcription. Tightly packed chromatin typically represses gene expression, while loosely packed regions allow for active transcription of genes.
- Epigenetic Modifications: These are chemical modifications to DNA and histones that affect how genes are expressed without altering the underlying DNA sequence.
Understanding genetic expression deepens our insight into how organisms develop and respond to their environment. This knowledge is instrumental in fields such as medicine, where understanding these mechanisms can lead to new therapeutic strategies.
Transcription Mechanism Overview
Transcription is a multi-step process involving several key events. Understanding this mechanism provides a clearer picture of how genetic information is used in biological systems.
Stages of Transcription:
- Initiation: The process begins when RNA polymerase binds to a specific region called the promoter. This binding causes the DNA strands to unwind and separate.
- Elongation: RNA polymerase traverses along the DNA template strand, synthesizing RNA in a 5' to 3' direction. During this phase, ribonucleotides are added to the growing RNA chain based on the complementary DNA template.
- Termination: Once a termination signal is reached, RNA polymerase detaches from the DNA, and the newly synthesized RNA strand is released.
"Understanding the detailed mechanism of transcription not only clarifies how genes are expressed but also helps identify potential targets for therapeutic interventions."
Factors Influencing Transcription Efficiency:
- Promoter Strength: Different promoters have varying capabilities to initiate transcription.
- Transcription Factors: These proteins bind to the promoter and other regulatory sites to enhance or repress transcription.
- Chromatin Accessibility: The physical structure of DNA impacts transcription.
By grasping these concepts of transcription, researchers can explore its broader implications, whether in developmental biology, pathology, or therapeutic development.
Key Enzymes in RNA Synthesis
The topic of key enzymes in RNA synthesis is essential to understand the process of converting DNA to RNA. Enzymes play a vital role in the transcription process, facilitating the synthesis of RNA from the DNA template. They determine the effectiveness and efficiency of RNA synthesis, impacting gene expression and, consequently, various biological functions.
RNA Polymerase Functionality
RNA polymerase is the central enzyme in the transcription process. It synthesizes RNA by catalyzing the polymerization of ribonucleotides complementary to the DNA strand. In eukaryotes, three main types of RNA polymerase exist: RNA polymerase I, which synthesizes ribosomal RNA; RNA polymerase II, responsible for messenger RNA synthesis; and RNA polymerase III, which produces transfer RNA and other small RNAs. Each polymerase has specific functions and regulatory mechanisms that determine its activity.
The functionality of RNA polymerase involves several critical steps:
- Binding: RNA polymerase binds to the promoter region of the DNA. This is essential for the initiation of transcription.
- Catalysis: It catalyzes the addition of ribonucleotides to the growing RNA strand based on the DNA template.
- Proofreading: RNA polymerase has mechanisms to correct errors during RNA synthesis, enhancing the fidelity of transcription.
Overall, RNA polymerase is crucial for accurate and efficient RNA synthesis, underscoring its importance in molecular biology.
Factors Affecting Enzyme Activity
Several factors influence the activity of RNA polymerase and other enzymes involved in RNA synthesis. Understanding these factors is vital for optimizing conditions for research and practical applications.
Some of the factors include:
- Temperature: Enzyme activity is sensitive to temperature. Optimal temperatures enhance RNA polymerase activity, while extreme temperatures can lead to enzyme denaturation.
- pH Level: Each enzyme has a specific pH range in which it performs best. Deviating from this range can significantly affect its activity.
- Concentration of Nucleotides: An adequate supply of ribonucleotides is essential for efficient RNA polymerization. Low concentrations can slow the process.
- Presence of Inhibitors or Activators: Certain molecules can inhibit or activate RNA polymerase activity. Understanding these interactions is crucial for controlling transcription.
In summary, the functionality of RNA polymerase and the various factors affecting enzyme activity must be well understood to explore RNA synthesis effectively in both research and biotechnology.
The ability to control RNA polymerase activity could have transformative implications for genetic research and therapeutic development.
For more information on enzymes and their functions, you can explore resources like Wikipedia and Britannica.
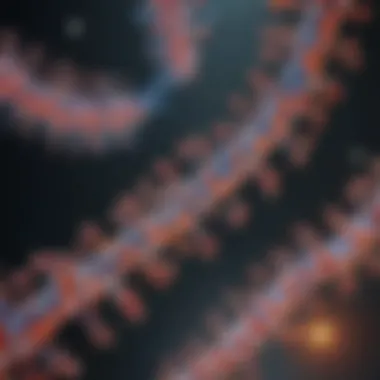
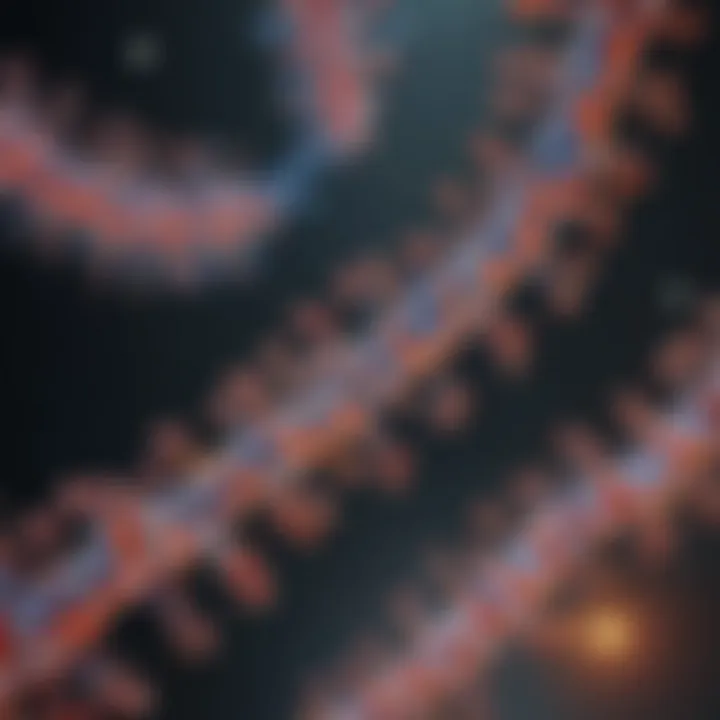
Mechanisms of DNA to RNA Conversion
The conversion of DNA to RNA is a critical process in the world of molecular biology. This process, known as transcription, serves as the bridge between genetic information stored in DNA and the functional proteins that drive cellular activities. Understanding the mechanisms at play in transcription is essential for both educational purposes and practical applications in research and biotechnology. Different elements of transcription, including initiation, elongation, and termination, contribute substantially to how genes are expressed and regulated.
Initiation of Transcription
The initiation phase marks the beginning of transcription. It requires the binding of RNA polymerase to a specific region of the DNA called the promoter. This region acts as a signaling area for the RNA polymerase, determining where to start copying the DNA sequence into RNA. The role of transcription factors is significant at this stage, as they assist in recruiting the RNA polymerase to the correct location.
Once bound, the DNA strands unwind, allowing access to the template strand. This is a crucial step, as the specific part of the DNA to be transcribed can vary depending on various cellular conditions and regulatory mechanisms. Understanding the precise mechanisms involved in this phase is vital for researchers aiming to manipulate gene expression.
Elongation Phase
Following initiation, the elongation phase continues the transcription process. The RNA polymerase enzyme moves along the DNA template strand, synthesizing a complementary RNA strand. This occurs in the 5' to 3' direction, adding ribonucleotides one by one. The enzyme unwinds the DNA ahead and rewinds it behind, ensuring that the transcription bubble forms and remains stable throughout this phase.
This stage is marked by the robustness of RNA synthesis, which can occur at a rapid pace. Errors in this phase, although relatively rare, can lead to mutations in the RNA, impacting the final protein product. Awareness of how elongation works and the factors that can influence its efficiency is crucial for scientists working with gene expression and RNA modifications.
Termination of Transcription
The termination phase concludes the transcription process. This step occurs when RNA polymerase encounters specific sequences in the DNA known as terminators. Once these sequences are recognized, transcription halts, and the RNA molecule is released. This phase is as important as the others, as it ensures that only complete and accurate RNA transcripts are synthesized.
Termination can involve additional proteins that help to detach the RNA polymerase from the DNA. Understanding this process provides insights into gene regulation and the control of RNA synthesis, both of which are essential for maintaining cellular functions efficiently.
In summary, grasping the mechanisms of DNA to RNA conversion allows for a deeper appreciation of molecular biology, helping elucidate the complex pathways that govern life at the cellular level. The knowledge gained here is foundational for various applications in biotechnology, genetic research, and therapeutic interventions.
Methods for Analyzing RNA Sequence
Understanding the methods for analyzing RNA sequences is crucial in molecular biology. These methods allow scientists to explore the intricate details of RNA structure and function. They contribute significantly to our knowledge in genetics, cell biology, and biotechnology. Accurate analysis of RNA sequences is essential for various applications such as gene expression studies and the development of therapeutic strategies.
Employing RNA analysis techniques enables researchers to decode the genetic messages transcribed from DNA. Accurate sequencing can unveil information about gene activity in different conditions, thus aiding in the discovery of biomarkers and potential therapeutic targets.
Sequencing Techniques Overview
Recent advancements in sequencing technologies have transformed the landscape of RNA analysis. These techniques vary in sensitivity, speed, and cost, each offering unique advantages.
Key sequencing methods include:
- Sanger Sequencing: This traditional method uses chain-terminating inhibitors during DNA synthesis. While it's reliable for shorter sequences, it is less efficient for large-scale RNA analyses.
- Next-Generation Sequencing (NGS): This high-throughput technique allows for simultaneous sequencing of millions of fragments. NGS is beneficial for revealing complex transcriptomes and making detailed comparisons between samples.
- RNA-Seq: A specific type of NGS focused on capturing the entire RNA content. This method provides insights into gene expression levels, alternative splicing, and novel transcripts.
- Single-Cell Sequencing: With the ability to analyze RNA from individual cells, this technique uncovers heterogeneity within populations, proving valuable in developmental biology and cancer research.
Each sequencing method presents its own set of advantages and limitations. Selecting an appropriate technique depends on specific research needs, including the desired throughput and resolution. Moreover, as technology advances, methods improve, becoming more cost-effective and accessible.
PCR Amplification for RNA
Polymerase Chain Reaction (PCR) is vital in RNA analysis. This technique enables amplification of specific RNA sequences, allowing for easier analysis and quantification. Before conducting PCR, it is essential to convert RNA into complementary DNA (cDNA) using reverse transcription. This conversion retains the necessary information from the original RNA.
PCR amplification for RNA involves several steps:
- Reverse Transcription: The initial step converts RNA into cDNA, a more stable form that can be amplified easily.
- Primer Design: Specific primers must be designed to target the sequence of interest. Primer specificity is crucial to avoid non-specific amplification.
- Amplification: The PCR process involves cycles of denaturation, annealing, and extension, enabling the exponential increase of the target DNA.
- Analysis: The amplified products can then be analyzed through methods such as gel electrophoresis or quantitative PCR (qPCR).
PCR plays a significant role in diagnostics, biomarker discovery, and research applications. Its ability to amplify RNA sequences allows detection of low-abundance transcripts with high specificity.
Applications of RNA Synthesis
The applications of RNA synthesis in science and technology are broad and impactful. RNA plays a crucial role in various biological processes, and understanding its synthesis opens pathways to ground-breaking applications in different fields. This section outlines significant areas where RNA synthesis is applied, underscoring the benefits and considerations accompanying each.
In Biotechnology
Biotechnology heavily relies on RNA synthesis for several applications. One notable aspect is the production of recombinant proteins. By utilizing messenger RNA (mRNA) derived from specific genes, researchers can translate these sequences into proteins in vitro. This approach facilitates drug discovery and production of therapeutic proteins.
Key benefits in biotechnology include:
- Increased efficiency: RNA synthesis allows for the rapid production of proteins without using whole cells.
- Precision: Scientists can modify the RNA sequence to create proteins with desired amino acids, tailored for specific functions.
- Cost-effectiveness: Synthetic RNA technology can reduce overall production costs compared to traditional methods.
RNA synthesis also finds applications in the development of RNA-based vaccines. The mRNA vaccines for COVID-19, such as the ones developed by Pfizer and Moderna, are prime examples. These vaccines use synthetic mRNA that instructs cells to produce a harmless piece of the virus, eliciting an immune response.
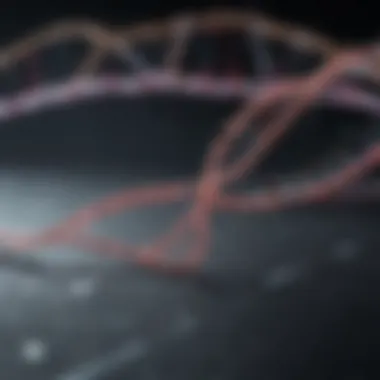
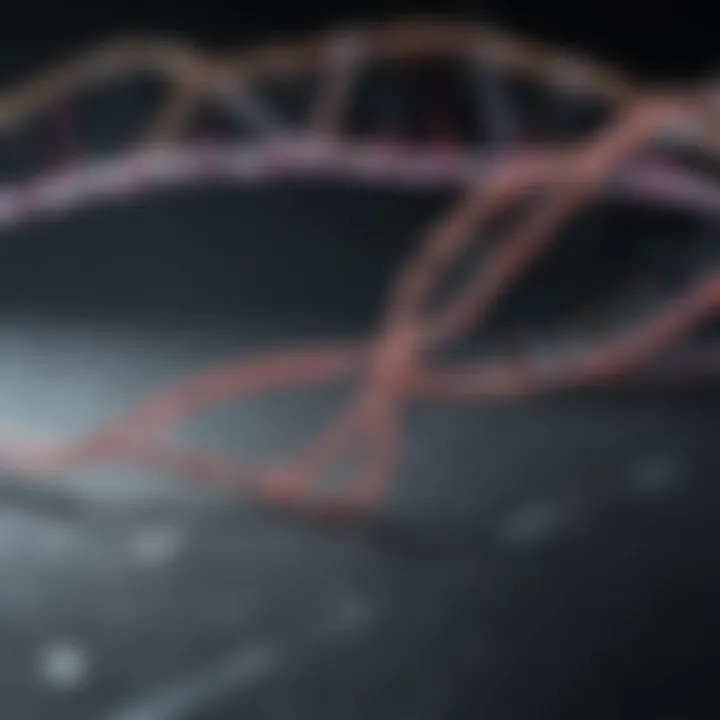
In Genetic Engineering
RNA synthesis is indispensable in the field of genetic engineering. It allows for precise manipulation of genes, supporting advancements in gene editing technologies. Techniques like CRISPR-Cas9 utilize RNA components to guide the editing process. The guide RNA (gRNA) directs the Cas9 enzyme to the targeted DNA sequence, facilitating accurate edits.
Additionally, RNA synthesis is vital for creating synthetic organisms. By designing specific RNA sequences, researchers can program cells to exhibit new traits or produce useful compounds. This application holds promise for addressing challenges such as sustainable agriculture and biofuel production.
Considerations in genetic engineering include:
- Safety: Genetic modifications can lead to unintended consequences in ecosystems if not managed properly.
- Ethics: There are ongoing debates about the implications of altering genetic material, particularly in human subjects.
Challenges in RNA Conversion
In the process of converting DNA into RNA sequences, several challenges can hinder efficiency and accuracy. Understanding these challenges is critical for researchers, educators, and students involved in molecular biology. As the field progresses, addressing these issues becomes increasingly important, especially in applications like biotechnology and genetic research.
One of the foremost challenges in RNA conversion is the occurrence of errors during transcription. These errors can stem from various factors, such as the fidelity of RNA polymerase during the recruitment of RNA nucleotides. Misincorporation of nucleotides can lead to nonfunctional or dysfunctional RNA, which disrupts the downstream processes such as translation into proteins. Furthermore, external factors like temperature changes and the presence of inhibitors can impact the transcriptional fidelity.
Another significant issue is the degradation of RNA after its synthesis. RNA molecules are inherently unstable and can undergo rapid degradation by enzymes called RNases. These enzymes are prevalent in biological systems and can degrade RNA even under mild conditions. Once RNA degrades, it results in a loss of information and can adversely affect experimental outcomes. Understanding the stability of RNA and implementing protective measures, such as storing RNA samples properly, play essential roles in managing this challenge.
"Being aware of the challenges in RNA conversion is crucial for improving methodologies and outcomes in molecular biology research."
The implications of these challenges extend not only to the laboratory practices but also to therapeutic development and other applications. Innovations aimed at mitigating transcription errors could enhance the quality of RNA products. Likewise, advancements in RNA preservation methods can ensure that synthesized RNA maintains its integrity for research and clinical use.
The following subsections will explore specific challenges in more depth, including the nature and causes of errors during transcription, as well as strategies to minimize RNA degradation. This knowledge is essential for developing robust techniques that enhance RNA synthesis accuracy and utility.
Future Directions in RNA Research
RNA research continues to evolve, encompassing a variety of exciting developments that promise to revolutionize our understanding of genetics and biology. As researchers push the boundaries of knowledge, they increasingly rely on innovative technologies and methodologies to explore RNA's role in cellular processes, disease mechanisms, and therapeutic applications. It is crucial to grasp the significance of these future directions in RNA research as they pave the way for breakthroughs in multiple fields.
Emerging Technologies
Emerging technologies in RNA research are transforming the landscape of molecular biology. Techniques like CRISPR-Cas9 for RNA-targeted gene editing offer unprecedented precision. This innovation allows scientists to modify RNA sequences with high accuracy, leading to important advancements in genetic studies and therapeutic interventions.
Other technologies, such as RNA sequencing, continue to advance rapidly. With high-throughput sequencing platforms, researchers can now analyze the transcriptome with remarkable detail. This capability enhances our understanding of gene expression patterns and their relation to various biological functions. Importantly, these technologies aid in detecting alternative splicing events, providing insights into cellular responses and complexities.
- High-throughput sequencing: Enables comprehensive analysis of RNA populations.
- CRISPR-Cas9 applications: Facilitates targeted editing of RNA sequences.
- Nanopore sequencing: Offers real-time analysis of RNA molecules, improving speed and efficiency.
This technological evolution not only supports basic research but also enhances diagnostic capabilities and therapeutic options in clinical settings.
Potential Therapeutic Applications
Researchers are increasingly exploring the therapeutic potential of RNA. One promising avenue involves the use of small interfering RNA (siRNA) and messenger RNA (mRNA) for gene therapy. siRNAs are designed to silence specific genes associated with diseases, including cancer. mRNA vaccines, such as those developed for COVID-19, highlight the potential for using RNA as a platform for rapid vaccine development.
Moreover, RNA-based therapeutics can directly target genetic disorders that arise from mutations, presenting a new frontier in personalized medicine. Some notable potential applications include:
- Oncology treatments: Utilizing RNA to silence oncogenes or enhance immune responses.
- Genetic disorder interventions: Correcting defective RNA transcripts to restore normal function.
- Vaccine development: Leveraging mRNA technology for rapid production of immune responses to pathogens.
These applications indicate a shift towards a more RNA-centric focus in therapeutic strategies, illustrating its immense potential.
"The future directions in RNA research not only refine our scientific understanding but also provide the foundation for transformative therapies in clinical practice."
As we move forward, the integration of these innovative technologies with a clear focus on therapeutic applications will likely reshape the landscape of molecular biology and medicine, making it an exhilarating time for RNA research.
Culmination
The conclusion of this article serves as a critical summary of the transformation from DNA to RNA sequences. This conversion is a fundamental biological process that underpins all genetic operations. Understanding this topic helps illuminate the complexity of molecular biology and its applications in various fields.
Summarizing Key Points
In reviewing the key elements discussed, we find several crucial points:
- Transcription Mechanism: The process of transcription is vital, as it is the method through which the genetic information encoded in DNA is transcribed into RNA. This process encompasses initiation, elongation, and termination phases, all of which are finely regulated.
- Role of Enzymes: Enzymes such as RNA Polymerase play a central role in synthesizing RNA from a DNA template. Their function can be influenced by various factors, emphasizing the dynamic and adaptable nature of transcription.
- Challenges: Errors can occur during transcription, leading to RNA degradation and inaccuracies in gene expression. Understanding these challenges is essential for improving techniques in research and biotechnology.
Clearly, the DNA to RNA process is not just a series of events, but a holistic mechanism integral to life itself. This interconnectedness makes it crucial for students, researchers, and educators to grasp its implications.
The Importance of Ongoing Research
Ongoing research in RNA biology is essential. As scientific understanding progresses, new insights into RNA functions, structures, and interactions emerge. These advances offer potential therapeutic applications that could lead to breakthroughs in healthcare and genetic engineering. Furthermore, emerging technologies continue to refine our ability to analyze RNA sequences, making research not only relevant but also imperative for future innovations.
In essence, a commitment to research in this area fosters an environment of discovery. It contributes to deeper comprehension of genetic expression and its manipulation, which can have profound effects on biotechnology and medicine. As we continue to explore these frontiers, we can redefine our approach to genetic diseases and develop novel therapeutic strategies.