From DNA to mRNA: Insights into Transcription
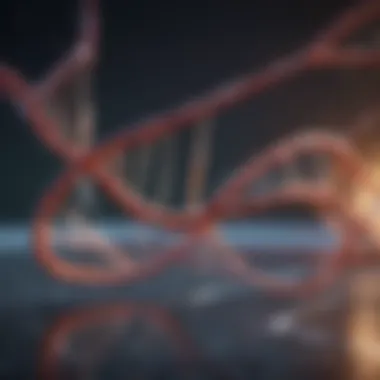
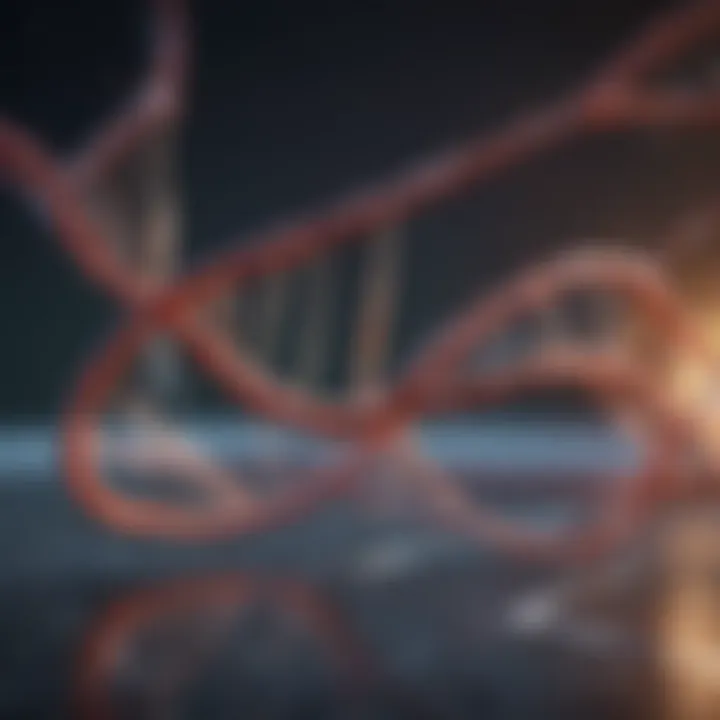
Intro
Transcription stands as a pivotal mechanism in the realm of molecular biology, marking the initial step in gene expression. This process involves the conversion of DNA sequences into messenger RNA (mRNA), which ultimately serves as a critical intermediary. Understanding this biological phenomenon is essential for both educational and professional pursuits in genetics and biotechnology. This overview aims to clarify the nuances of transcription, the enzymes that facilitate it, and its implications for cellular functions and medical advancements.
The intricate nature of transcription showcases how genetic information is transferred and utilized within cells. Each phase, from initiation to elongation and termination, unfolds layers of complexity, engaging a variety of biomolecules. The significance of mRNA extends beyond serving as a template for protein synthesis; it plays a vital role in various cellular processes and has been harnessed in biotechnological applications, such as mRNA vaccines, which gained notoriety during the COVID-19 pandemic.
Through this article, we will meticulously examine:
- The stages of transcription
- The enzymes involved, particularly RNA polymerase
- The influence of transcription factors
- Post-transcriptional modifications of mRNA
- The broader implications of mRNA in healthcare and research.
In recognizing the ongoing advances in gene expression studies, future research directions will also be considered. Each of these aspects contributes to a comprehensive understanding of how life at a molecular level unfolds.
Preamble to DNA and mRNA
Understanding the relationship between DNA and mRNA is fundamental in molecular biology. This knowledge offers insights into how genetic information is expressed and regulated within a cell. DNA, or deoxyribonucleic acid, serves as the blueprint of life, encoding the specific instructions required for an organism's development and functioning. Conversely, messenger RNA, or mRNA, acts as the intermediary that conveys these instructions from the DNA to the cellular machinery responsible for protein synthesis.
The significance of this transformation process cannot be overstated. In simple terms, it is through transcription that the information stored in the DNA is converted into a form that can be utilized by cells to produce proteins. These proteins are essential for a myriad of functions, including structure, function, and regulation of tissues and organs. Furthermore, any alterations in this process can lead to significant biological consequences, making the understanding of transcription vital for fields such as genetics, biotechnology, and medicine.
Fundamentals of DNA Structure
DNA is composed of two strands that coil around each other to form a double helix. The basic units of DNA are nucleotides, which consist of three components: a phosphate group, a sugar molecule (deoxyribose), and a nitrogenous base. There are four types of nitrogenous bases found in DNA: adenine (A), thymine (T), cytosine (C), and guanine (G).
The sequence of these bases encodes genetic information. The arrangement of these bases along the DNA strand determines the genetic instructions carried by that segment of DNA. Each set of three nucleotides, known as a codon, correlates to a specific amino acid, the building blocks of proteins. This precise structure enables DNA to replicate itself and serve as a template for mRNA synthesis.
Role of mRNA in Protein Synthesis
mRNA serves as the critical link between DNA and protein synthesis. During transcription, mRNA is synthesized from a DNA template. This process involves the copying of genetic information from the DNAβs coding strand into a complementary RNA sequence. mRNA then carries this information from the nucleus to the cytoplasm, where it guides the synthesis of proteins at the ribosome.
mRNA is vital for accurate gene expression. An mRNA molecule carries the specific codes needed for assembling amino acids in the correct order to form functional proteins. Various factors can influence this process, including mRNA stability, localization, and degradation, which can directly impact cellular function and a host of biological processes.
In summary, grasping the fundamental concepts of DNA structure and the role of mRNA is crucial for a deeper understanding of the transcription process. This knowledge lays the groundwork for exploring how genes are expressed and regulated within biological systems.
The Basics of Transcription
Understanding the basics of transcription is essential for comprehending the fundamental processes that govern gene expression. This section outlines the significance of transcription, where the genetic information encoded in DNA is transcribed into messenger RNA (mRNA). By grasping this process, researchers and students can appreciate a pivotal step in molecular biology that is crucial for cellular function.
Definition and Importance
Transcription is the process whereby a specific segment of DNA is copied into RNA by the enzyme RNA polymerase. This is a crucial step in the flow of genetic information from DNA to proteins, which ultimately affect cellular functions.
The importance of transcription lies not only in its fundamental role in gene expression, but also in the regulation of biological processes. It enables cells to produce the right proteins at the right time, in response to various signals. Without transcription, cellular activities would be severely hampered, leading to potential dysfunctions in organismal development and responses to the environment.
Key Components of Transcription
Several key components are involved in the transcription process, making it a complex yet well-orchestrated event. Some of these components include:
- RNA Polymerase: This enzyme is responsible for synthesizing RNA. It unwinds the DNA double helix and assembles RNA nucleotides into a complementary strand.
- Promoter Regions: Located upstream of the gene, these regions contain specific sequences that signal when to initiate transcription. They determine where transcription starts and often regulate the transcription's intensity.
- Transcription Factors: These protein molecules bind to specific DNA sequences, facilitating or hindering the binding of RNA polymerase to the promoter. They play vital roles in controlling gene expression patterns.
- Elongation Factors: These factors assist RNA polymerase during the elongation phase, ensuring efficient RNA synthesis.
In summary, understanding the basics of transcription is vital for appreciating how genes are expressed and regulated within the cell. This knowledge serves as a foundation for deeper exploration into gene function, cellular processes, and advanced biotechnological applications.
Transcription Factors and Their Functions
Transcription factors are essential proteins that play a critical role in the regulation of gene expression. Their importance cannot be understated, as they serve as critical components in the transcription process. They bind to specific DNA sequences and enable or inhibit the transcription of target genes. Without the precise actions of transcription factors, the entire process of turning DNA into mRNA could falter, leading to significant cellular malfunction.
Overview of Transcription Factors
Transcription factors can be classified into two main categories: general transcription factors and specific transcription factors. General transcription factors are necessary for the transcription of all genes and are involved in the basic machinery of the transcription process. They help RNA polymerase to bind to the promoter region of DNA.
Specific transcription factors, on the other hand, are tailored to regulate specific genes. They may respond to external signals, which allows cells to adapt based on their environment. Thus, transcription factors are integral not only in facilitating the process of transcription but also in ensuring that genes are activated or silenced at the right times.
Transcription factors exhibit diverse mechanisms due to their structure. Many have domains that recognize and bind to specific DNA sequences, often referred to as enhancer or silencer regions. These regions can be located far from the gene they regulate. Consequently, transcription factors can exert their influence over large genomic distances, highlighting their importance within the broader context of gene regulation.
How Transcription Factors Regulate Gene Expression
Transcription factors regulate gene expression through several mechanisms. Here are some of the key ways they function:
- Binding to DNA: They attach to specific sites on the DNA, usually in the promoter region. This binding can either promote or inhibit RNA polymeraseβs ability to initiate transcription.
- Recruitment of Co-factors: Transcription factors can recruit additional proteins that assist in the transcription process. These co-factors can help modify chromatin structure, thus opening or closing the DNA for transcription.
- Signal Integration: They can integrate signals from various pathways, allowing the cell to respond dynamically to changes in the environment. This is essential for processes like cell differentiation.
- Transcriptional Activation or Repression: Depending on the context, transcription factors can facilitate the recruitment of RNA polymerase to promote gene expression or prevent polymerase binding to repress a gene.
"Transcription factors act as gatekeepers of gene expression, determining when and how much of a gene product is made."
Understanding these functions is crucial for comprehending how cells control their inner workings. Moreover, misregulation of transcription factors can lead to various diseases, including cancer. Thus, studying transcription factors represents an important frontier in both basic biology and disease research.
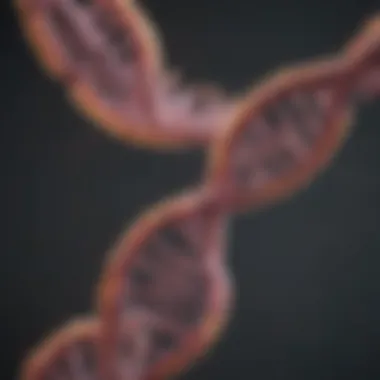
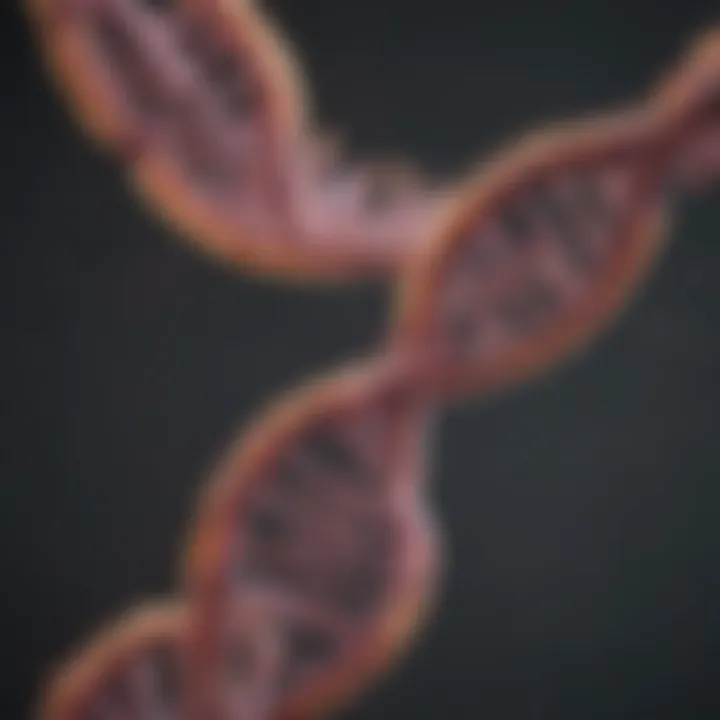
The Role of RNA Polymerase
RNA polymerase is an essential enzyme responsible for synthesizing RNA from a DNA template during the transcription process. This enzyme plays a crucial role in gene expression by catalyzing the formation of mRNA, which is integral to the translation of genetic information into proteins. The function of RNA polymerase highlights not just its enzyme activity but also its interactions with various factors that modulate transcription.
By understanding RNA polymerase, researchers can gain insights into how the genetic code is accessed and utilized within cells. Its significance is underscored by the various types of RNA polymerases present in different organisms and their specialized functions. Moreover, RNA polymerase is also a target for many therapeutic interventions, illustrating its importance in biotechnology.
Types of RNA Polymerase
There are several types of RNA polymerase, each with distinct functions:
- RNA Polymerase I: This type primarily synthesizes ribosomal RNA (rRNA), which is a critical component of ribosomes, the cellular machinery for protein synthesis.
- RNA Polymerase II: This enzyme is responsible for synthesizing messenger RNA (mRNA) and some small nuclear RNAs (snRNA). It is the most studied polymerase due to its direct role in protein-coding gene expression.
- RNA Polymerase III: This enzyme synthesizes transfer RNA (tRNA), along with some other small RNA molecules that play roles in protein synthesis and gene regulation.
Each type of RNA polymerase works at different types of promoter regions specific to their functions, ensuring that the correct RNA molecules are synthesized at the right time and location within the cell.
Mechanism of mRNA Synthesis
The mechanism of mRNA synthesis by RNA polymerase is a multi-step process that includes initiation, elongation, and termination. During the initiation phase, RNA polymerase binds to promoter regions on the DNA, aided by transcription factors that help position the enzyme correctly. Once bound, RNA polymerase unwinds the DNA double helix to expose the coding strand, making it accessible for transcription.
As transcription progresses to the elongation phase, RNA polymerase traverses down the DNA template strand, catalyzing the addition of ribonucleotides to the growing mRNA strand. This process occurs in a 5' to 3' direction, synthesizing a complementary strand based on the DNA sequence. The enzyme moves along the DNA, maintaining a transcription bubble where the strands are separated.
Finally, termination of transcription occurs when RNA polymerase encounters specific sequences known as termination signals. The enzyme detaches from the DNA, resulting in the release of the newly synthesized mRNA transcript. This mRNA will undergo further processing before being utilized in the translation of proteins.
Understanding the structural and functional aspects of RNA polymerase is vital for deciphering gene expression and its regulation in health and disease.
Initiation of Transcription
The initiation of transcription is critical in the process of gene expression. This phase sets the stage for RNA synthesis from the DNA template. In essence, it determines which genes are activated, influencing the cellular functions and overall behavior of an organism. Her understanding the intricacies of this process is fundamental for researchers and professionals in the fields of molecular biology and genetics.
Promoter Regions
Promoter regions are specific sequences in the DNA that signify where transcription begins. They are located upstream of the gene they regulate. These regions serve as binding sites for transcription factors and RNA polymerase, crucial elements necessary for the initiation process. Each promoter is characterized by unique sequences that can enhance or inhibit the binding of these vital components.
Understanding these sequences is beneficial, as it allows scientists to manipulate gene expression. For instance, in genetic engineering, researchers may use specific promoter sequences to activate or silence genes. The classic TATA box is a well-studied example. Located approximately 25-30 base pairs upstream of the transcription start site, the TATA box provides a platform for RNA polymerase II to initiate mRNA synthesis.
Assembly of the Transcription Complex
The assembly of the transcription complex is a multi-step process that requires precise interactions between various protein factors and DNA sequences. Firstly, transcription factors bind to the promoter regions, creating a foundation for RNA polymerase to attach. These factors not only help in recruiting RNA polymerase but also play roles in stabilizing the entire complex. They ensure RNA polymerase begins at the correct site on the DNA.
Once the transcription factors are bound, RNA polymerase joins the assembly, forming the pre-initiation complex. This stage is vital as it marks the transition from a dormant gene to an actively transcribing one.
The successful assembly of this complex means that the necessary signals for gene activation are in place. Researchers are exploring how disruptions in this assembly can lead to various diseases, demonstrating the importance of understanding this initiation phase.
"The precision of transcription initiation determines the fate of cellular activity and the organism itself."
In summary, the initiation of transcription plays a pivotal role in gene regulation and expression. A thorough understanding of promoter regions and the assembly of the transcription complex allows for insights into molecular mechanisms governing cell function, paving the way for advancements in biotechnology and medicine.
Elongation Phase of Transcription
The elongation phase of transcription is pivotal in the pathway from DNA to mRNA. This is where the actual synthesis of the RNA strand takes place. As RNA polymerase continues from the initiation stage, it unwinds the DNA strands and synthesizes the complementary RNA strand, thus transcribing the genetic code. Understanding this phase is crucial for grasping how genes are expressed and how proteins are subsequently synthesized.
During elongation, RNA polymerase moves along the DNA template, adding ribonucleotides to the growing RNA chain in a sequence complementary to the DNA. This behavior confirms that the information encoded in the DNA is being effectively translated into a form that can be utilized for protein synthesis. The elongation phase not only marks a significant step in transcription but also has implications in gene regulation and expression.
Mechanisms of RNA Elongation
The mechanisms involved in RNA elongation are intricate yet streamlined. Once the transcription complex is assembled, RNA polymerase catalyzes the elongation of the RNA molecule. This occurs in a specific manner:
- Nucleotide Addition: The enzyme RNA polymerase adds nucleotides to the 3β end of the RNA molecule. The ribonucleotides are paired with their complementary DNA bases, following the rule of base pairing.
- Direction of Synthesis: The production of mRNA occurs in the 5β to 3β direction. This means that RNA polymerase reads the DNA template in the 3β to 5β direction, facilitating the continuous addition of nucleotides.
- Polymerase Movement: Movement of RNA polymerase along the DNA strand is facilitated by the unwinding of the DNA helix. The RNA polymerase travels multiple nucleotides ahead of the elongation site, allowing the DNA to re-anneal after RNA has been synthesized.
- Error Correction: Although RNA polymerase has a relatively high fidelity, errors can occur during transcription. The enzyme possesses a proofreading ability, allowing it to correct mistakes in real time to ensure the accuracy of the mRNA strand.
Modifications During Elongation
Modifications during the elongation phase are critical for the maturation of mRNA. These changes help in the stability and functionality of the mRNA. Two prominent modifications include:
- Capping: At the 5β end of the growing RNA strand, a special guanine nucleotide is added. This 5β cap protects the mRNA from degradation and is essential for the initiation of translation.
- Polyadenylation: After elongation, a stretch of adenine nucleotides (poly-A tail) is added to the 3β end. This poly-A tail serves several purposes: it enhances the stability of the mRNA and aids in the export of mRNA from the nucleus to the cytoplasm.
These modifications are crucial because they ensure that mRNA fulfills its role in protein synthesis efficiently.
Understanding the elongation phase of transcription sheds light on how genetic information is converted into functional molecules in the cell, setting the stage for subsequent processes that lead to protein synthesis.
Termination of Transcription
Termination of transcription marks a crucial final step in the process of converting DNA sequences into mRNA. This phase ensures that the RNA polymerase enzyme, after synthesizing a complementary RNA strand, disengages properly from the DNA template. The specifics surrounding termination are vital because they affect the accuracy and functionality of the resulting mRNA. An essential consideration is the preservation of the genetic information during transcription. Failure to terminate correctly can lead to longer or defective RNA transcripts, which may cause errors in subsequent protein synthesis.
The termination process also signifies the end of gene expression and the readiness of the mRNA molecule for post-transcriptional modifications. Understanding this process is critical, especially for those working in fields such as molecular biology and genetics. In this section, we will explore the details of termination, covering termination signals and the eventual release of the mRNA transcript.
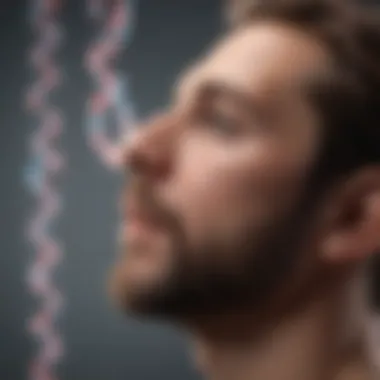
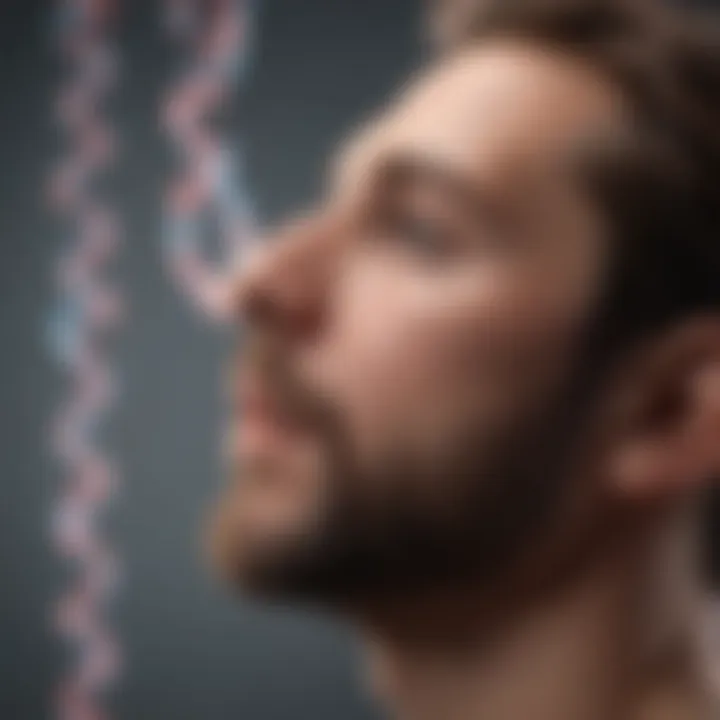
Termination Signals in DNA
Termination signals are specific sequences within the DNA that indicate to RNA polymerase where to stop transcription. These sequences can vary among different organisms, but they generally share similar features. In eukaryotes, there are two primary types of termination signals:
- Intrinsic (or Rho-independent) signals: These are sequences that form hairpin structures in the RNA, causing the polymerase to pause and ultimately detach. This structural formation leads to the instability of the RNA-DNA hybrid and promotes the release of the newly synthesized mRNA.
- Rho-dependent signals: These require a protein known as Rho factor. After RNA polymerase synthesizes the mRNA, the Rho protein attaches to the growing RNA strand and moves towards the polymerase. Upon reaching the enzyme, Rho causes it to dissociate from the DNA template, thereby completing the transcription process.
These termination signals are essential for the precise regulation of gene expression. Inadequate recognition or malfunction of these signals can lead to aberrant transcription, producing non-functional mRNA that could disrupt cellular processes.
Release of mRNA Transcript
Once the RNA polymerase encounters termination signals, the next critical step is the release of the mRNA transcript. This release is influenced primarily by the structural changes that the newly formed mRNA undergoes after transcription. The significance of this release process cannot be overstated, as it determines the availability of mRNA for subsequent stages of gene expression, including translation.
During this phase, the polymerase enzyme must separate from the mRNA and the DNA template. This separation is not merely a mechanical process; it involves intricate biochemical interactions that govern the stability and structure of the mRNA strand.
Following release, the newly formed mRNA is subject to various post-transcriptional modifications, which enhance its stability and usability. The cap structure added to the 5β end and the poly-A tail at the 3β end are critical modifications that play important roles in protecting the mRNA from degradation and facilitating its export from the nucleus to the cytoplasm.
In summary, the termination of transcription is intricately connected to the accuracy and regulation of mRNA production. Learning about termination signals and the release mechanisms provides insight into critical biological processes and the complexity of gene expression.
Post-Transcriptional Modifications of mRNA
After the synthesis of mRNA from DNA during transcription, the resulting molecule undergoes several essential modifications. These modifications play a crucial role in stabilizing the mRNA, facilitating its translation into proteins, and regulating its function within the cell. Without these post-transcriptional modifications, mRNA would be less effective in guiding protein synthesis, leading to potential cellular dysfunction. This section will elucidate the specific modifications that occur and their significance.
Capping and Polyadenylation
Capping is the first major modification that occurs on the mRNA molecule shortly after transcription begins. A unique structure called the 5' cap is added to the beginning of the mRNA transcript. This cap protects mRNA from degradation by nucleases and assists in the initiation of translation. The cap provides a binding site for ribosomes, ensuring that the mRNA is efficiently translated into its corresponding protein.
Following capping, a poly(A) tail is added to the 3' end of the mRNA. This tail consists of a long chain of adenine nucleotides and serves multiple functions:
- Stabilization: The poly(A) tail significantly increases the stability of the mRNA molecule, protecting it from enzymatic degradation.
- Nuclear Export: It helps facilitate the export of mRNA from the nucleus to the cytoplasm where translation occurs.
- Translation Efficiency: The poly(A) tail is also crucial for the recognition of mRNA by the ribosome, enhancing the efficiency of translation.
Both capping and polyadenylation are vital for the life and utility of mRNA within the cell. They ensure that the mRNA remains intact long enough to fulfill its role in protein synthesis.
Splicing Mechanisms
Splicing is another critical post-transcriptional modification that occurs in eukaryotic cells. During this process, non-coding sequences called introns are removed from the pre-mRNA transcript, while coding sequences, known as exons, are joined together. This splicing is performed by a complex known as the spliceosome, which comprises various proteins and RNA molecules.
The importance of splicing cannot be understated:
- Functional Diversity: By allowing different combinations of exons to be joined together, splicing produces multiple mRNA isoforms from a single gene. This phenomenon is known as alternative splicing and plays a significant role in increasing the diversity of proteins that can be synthesized.
- Regulation of Gene Expression: Splicing can also regulate gene expression levels. By selectively including or excluding certain exons, the cell can modulate which proteins are produced and in what amounts.
"Splicing provides a mechanism for generating protein diversity, allowing organisms to adapt and evolve."
In summary, post-transcriptional modifications of mRNA, including capping, polyadenylation, and splicing, are essential processes that enhance the stability, functionality, and versatility of mRNA. These modifications are not mere afterthoughts; they are integral to the effective expression of genes and the overall cellular processes. Understanding these modifications opens new avenues in the study of molecular biology and genetics.
The Journey of mRNA to the Ribosome
The process of transcription concludes with the synthesis of mRNA, but this is only the beginning of mRNA's critical role in protein synthesis. The journey of mRNA to the ribosome is a vital step that ensures the translation of genetic information into functional proteins. This section details the mechanisms involved in mRNA export from the nucleus and provides an overview of the translation process itself. Understanding these steps helps to clarify the importance of mRNA within cellular life.
mRNA Export from the Nucleus
Once mRNA is synthesized in the nucleus, it must undergo a series of modifications before it can travel to the cytoplasm. This export process is essential for the mRNA to be functional. Several key elements are involved in this phase:
- Nuclear Pore Complex: The mRNA is transported through the nuclear pore complex. This structure acts like a gatekeeper, allowing only properly processed mRNA to enter the cytoplasm.
- Capping and Polyadenylation: Before export, mRNA is capped at the 5' end and polyadenylated at the 3' end. These modifications not only enhance stability but also signal that the mRNA is ready to be translated.
- Export Proteins: Specific proteins, such as exportin, bind to the mRNA and facilitate its translocation out of the nucleus. These proteins are crucial for recognizing the modified mRNA and escorting it through the pore complexes.
The movement of mRNA from the nucleus to the cytoplasm is a tightly regulated process. Errors in this export can lead to defects in protein synthesis and various cellular dysfunctions.
Translation Process Overview
Upon reaching the cytoplasm, mRNA is translated into proteins through a process involving ribosomes and transfer RNA (tRNA). This translation process can be summarized in several important steps:
- Initiation: The small subunit of the ribosome binds to the start codon on the mRNA. This is crucial as it sets the reading frame for the sequence to be translated.
- tRNA Matching: Transfer RNA molecules, charged with specific amino acids, recognize and bind to complementary codons on the mRNA. The tRNA acts as an adaptor, translating the genetic code into a polypeptide chain.
- Elongation: The ribosome moves along the mRNA, and tRNAs sequentially add amino acids to the growing chain, which folds into a functional protein. Each elongation cycle is a precise process, ensuring that the sequence is followed correctly.
- Termination: Translation ends when a stop codon is reached. Release factors help detach the newly synthesized protein, and the ribosome subunits disassemble.
"The translation process is not just a simple reading of mRNA; it is a complex interplay of molecules that contributes to the diversity of proteins in living organisms."
This detailed process highlights the importance of mRNA not just as an intermediary of genetic information, but as a fundamental component of the cellular machinery that ultimately defines the functions of each cell. Understanding these mechanisms provides insight into both normal cellular processes and potential therapeutic applications.
The Role of mRNA in Cellular Processes
mRNA plays a vital role in the regulation of cellular processes. It is the intermediary between the genetic information encoded in DNA and the synthesis of proteins, which perform essential functions within the cell. This connection highlights the significance of mRNA in contributing to gene expression and its broader impact on cellular activities.
mRNA and Gene Expression Regulation
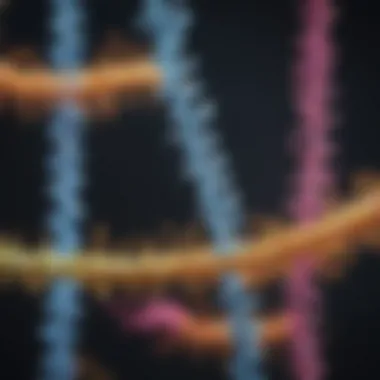
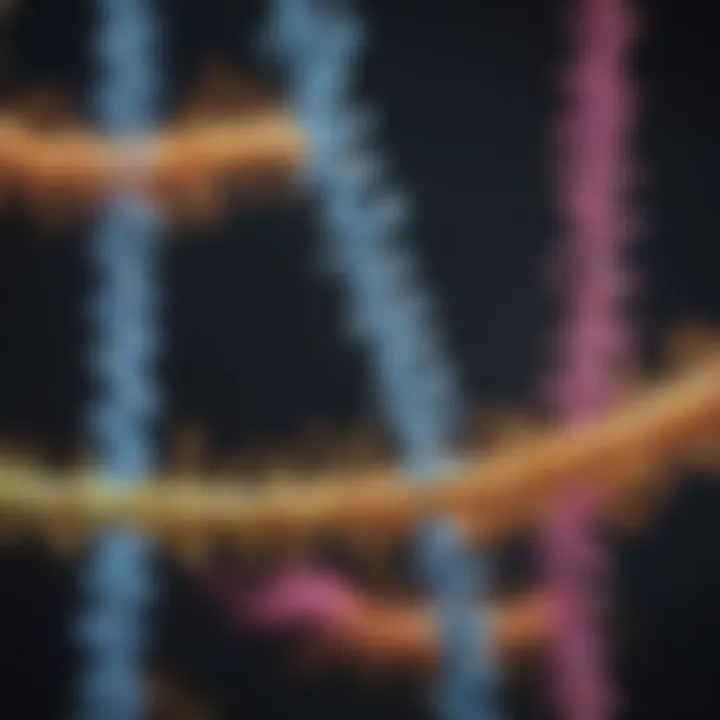
Gene expression regulation is fundamental in ensuring that specific genes are activated or suppressed based on the cell's needs. mRNA is central to this process because it carries the instructions from DNA that dictate protein synthesis.
Different factors influence mRNA's role in regulating gene expression, including:
- Transcription factors: These proteins bind to specific DNA sequences and can enhance or inhibit the transcription of mRNA.
- Splicing: This allows the production of different protein variants from a single gene, affecting which mRNA transcripts are available for translation.
- Stability: The half-life of mRNA in the cytoplasm influences how much protein can be synthesized from it. mRNAs that are more stable tend to be translated more frequently.
mRNA acts not only as a template for protein synthesis but also as a regulatory molecule that helps control the levels and timing of protein expression.
This regulation is crucial in processes like cell differentiation, development, and response to environmental signals. Understanding how mRNA contributes to these aspects can provide insights into various cellular functions and potential pathologies when these processes go awry.
Effects of mRNA on Cellular Function
The impact of mRNA on cellular function cannot be understated. By directing protein synthesis, mRNA influences a range of cellular activities, including:
- Metabolism: Specific mRNA molecules encode enzymes that regulate metabolic pathways.
- Cell growth and division: mRNA levels must align with cell cycle stages to ensure proper division and growth.
- Signal transduction: Many signaling proteins are synthesized in response to external stimuli, and their synthesis depends on available mRNA.
In future studies, understanding how to manipulate mRNA pathways could lead to new therapeutic strategies for diseases characterized by dysfunctional gene expression.
Biotechnological Applications of mRNA
The exploration of mRNA has uncovered significant potential in various biotechnological applications. Its role extends beyond mere transcription; it serves as a vital tool in modern medicine and other fields. This section will discuss the contributions of mRNA to vaccine development and its applications in therapeutic interventions. These applications exemplify how mRNA is leveraged to address pressing health challenges.
mRNA in Vaccines
A key application of mRNA technology is its use in vaccines. The most prominent example is the COVID-19 vaccines developed by Pfizer-BioNTech and Moderna. These vaccines utilize lipid nanoparticles to deliver synthetic mRNA to cells, which then produce a harmless piece of the virus's spike protein. This process trains the immune system to recognize and combat the real virus if encountered later. The efficacy of these mRNA vaccines has demonstrated rapid development timelines, which stands in contrast to traditional vaccine methods.
- Key Benefits:
- Rapid development and deployment in response to emerging infectious diseases.
- Strong immune response with fewer doses required compared to traditional vaccines.
- Flexibility in design allow for quick modifications against new variants.
Despite the success, there are considerations regarding production scalability and the need for proper storage conditions, which must be taken into account for widespread vaccination efforts.
Potential in Therapeutic Interventions
The promise of mRNA extends into therapeutic interventions, especially in the treatment of genetic diseases and cancer. By delivering mRNA that encodes therapeutic proteins, it may be possible to address the root causes of diseases at the molecular level. For example, mRNA therapies can be used to replace defective proteins or enhance the production of proteins that are critical for cell function.
- Applicable Area:
- Development of mRNA therapies for rare genetic disorders: Such interventions could provide patients with a viable treatment option when traditional medicines fail.
- Oncological applications: mRNA-based Cancer vaccines aim to stimulate the immune system to target and destroy cancer cells.
While the advancements in mRNA technology are promising, challenges remain, such as potential immune responses against the delivered mRNA, ensuring stability during delivery, and achieving efficient cellular uptake.
"The potential of mRNA is not only changing vaccine development but also transforming how we approach numerous therapeutic avenues."
As research continues, mRNA technologies may redefine multiple aspects of healthcare, marking a significant shift in how diseases are treated and prevented.
Challenges and Future Directions in mRNA Research
Understanding the transcription process is critical for comprehending mRNA's role in biology. However, this field faces various challenges that impact research efforts and applications. Addressing these obstacles not only enhances current studies but also paves the way for future advancements in the understanding of mRNA and its implications, particularly in biotechnology and medicine.
Current Limitations in mRNA Studies
While significant progress has been made in mRNA research, several limitations persist:
- Stability and Degradation: mRNA molecules can be unstable in cellular environments. Rapid degradation can hinder studies regarding mRNA lifespan and its functional impact, contributing to incomplete knowledge of gene expression regulation.
- Full-Length mRNA Analysis: Techniques like RT-PCR often do not account for the complete transcript. This limitation creates gaps in understanding alternative splicing events and isoform expression.
- Limited Methods for Structural Analysis: Current methodologies often fail to provide detailed insights into the three-dimensional structures that influence mRNA interactions and functionalities. This restricts practical applications in drug design and therapeutic interventions.
- In vivo vs. in vitro Studies: There is often a discrepancy between results obtained in laboratory settings and those in live organisms. This gap complicates the translation of findings to clinical applications.
"Understanding the stability and behavior of mRNA in vivo is crucial for developing effective therapies."
Emerging Research Trends
Despite these limitations, several exciting trends are emerging in mRNA research:
- mRNA Engineering: Scientists are now focusing on modifying mRNA molecules to enhance their stability and translation efficiency. This engineering could lead to improved therapies, particularly in vaccine development.
- Single-Cell RNA Sequencing: Advancements in this area allow for analyzing gene expression at the single-cell level. This provides deeper insights into cellular heterogeneity and the role of mRNA in various biological processes.
- Synthetic Biology Applications: Researchers are leveraging synthetic biology to design novel mRNA constructs. These can be tailored for specific therapeutic uses, expanding treatment options for different diseases.
- CRISPR-Cas9 Integration: The fusion of mRNA research with CRISPR technology is paving new pathways in gene editing and regulation, improving precision in genetic modification.
- Vaccine Development Innovations: The global focus on mRNA vaccines during health crises has stimulated research. This trend is likely to lead to rapid developments in immunization strategies beyond infectious diseases.
In summary, while challenges in mRNA research remain significant, ongoing discoveries and emerging trends provide a hopeful perspective for future advancements. Innovating through these hurdles is essential for unlocking the full potential of mRNA in therapeutic applications.
Ending
The conclusion of this article underscores the critical role mRNA plays in the transcription process. Understanding how DNA sequences are interpreted into mRNA is not just an academic exercise; it is foundational to fields such as molecular biology, genetics, and biotechnology.
Summary of Key Points
- Transcription Process: Involves the conversion of the genetic code from DNA to mRNA, facilitated by enzymes such as RNA polymerase.
- Key Components: Transcription factors and various regulatory elements are essential in initiating and controlling gene expression.
- Post-Transcriptional Modifications: These include capping, polyadenylation, and splicing, which prepare the mRNA for translation and influence its stability and activity.
- mRNA in Cellular Processes: Correct synthesis and modification of mRNA are vital for proper protein production, which is crucial for cell function and response to environmental changes.
- Biotechnological Applications: Research and application of mRNA have revolutionized vaccine development and have potential for therapeutic approaches.
The Ongoing Significance of mRNA Research
mRNA research continues to evolve, particularly after advancements highlighted during studies of COVID-19 vaccines. The mRNA technology has shown potential not just for vaccines but for gene therapy as well. Researchers are exploring how to improve mRNA stability and delivery mechanisms to enhance therapeutic effects. Furthermore, as scientists deepen understanding of mRNA regulation at cellular levels, new opportunities for treatments of genetic disorders and personalized medicine emerge.
Overall, the significance of mRNA research extends beyond academic interest. It holds the promise of advancing health sciences and creating innovative treatments through enhanced understanding of the transcription process.